Internal Nutrient Loading Controls Macroalgal and Cyanobacterial Succession in a Coastal Lagoon Restored by Managed Realignment of Agricultural Land
- Department of Biology, University of Southern Denmark, Odense, Denmark
Managed realignment (MR) has been increasingly applied as an adaptation strategy to sea level rise in low-lying coastal areas, but the ecological consequences after flooding agricultural land with seawater are not well known. The restored Gyldensteen Coastal Lagoon represents one of the largest MR projects in Europe to date. The area served as agricultural land for about 150 years before being deliberately flooded with seawater in 2014. This study monitored for 5 years the succession of macroalgae and benthic cyanobacteria driven by changing internal nutrient (DIN = NH4+ + NO2– + NO3–, DON = dissolved organic nitrogen, and DIP = PO43–) loadings in the lagoon after flooding. A massive bloom of opportunistic green macroalgae (dominated by Cladophora spp.) occurred during the first year as response to a substantial loading of DIN and DIP from the newly flooded soils. The macroalgal cover was sparse the following years and the species richness increased with lower loading of particularly DIN. A cyanobacterial bloom controlled by declining DIN and steady DIP concentrations in the water dominated the lagoon and covered all solid surfaces 4 years after flooding. Highest macroalgal species richness with dominance of perennial Fucus vesiculosus and Agarophyton vermiculophylla was recorded 5 years after flooding following a temperature-induced stimulation of soil nitrogen transformation, leading to increased water column DON concentrations and DIN:DIP ratios. The lagoon remains therefore at an unstable tipping point where small and random changes in the DIN:DIP ratio control the balance between blooms of benthic cyanobacteria and high macroalgal species richness. Future MR projects involving agricultural land should prepare the soil to prevent algal blooms driven by sustained internal nutrient loading. Particularly P loading should be avoided to minimize the chances for recurrent blooms of benthic cyanobacteria.
Introduction
Sea level rise due to climate change is now apparent along coastlines and is expected to continue at an accelerating pace in the decades to come (Nicholls and Cazenave, 2010; IPCC, 2019). This poses a flooding threat to low lying coastal areas among which many are embanked and reclaimed agricultural land (Nicholls and Cazenave, 2010). Maintaining protective defenses is expensive (e.g., Bosello et al., 2012; Hinkel et al., 2014), and coastal retreat by natural processes or through managed realignment (MR) is an alternative solution with the potential to restore otherwise lost coastal ecosystems (Boorman and Hazelden, 2017; Carey, 2020). Since the long-term ecological development after flooding agricultural land with seawater is almost unknown, there is a need for new research corroborating the development of best restoration practices and management of coastal areas threatened by sea level rise (Gerwing et al., 2020).
Coastal restoration by MR is typically initiated by constructing new inland dikes before a specific area is deliberately flooded by breaching the old seaward dikes (Esteves, 2014; Warner et al., 2018). The newly flooded area not only acts as a buffer to reduce physical forcing by, e.g., wave action on the inland areas but also creates new coastal habitats for colonization by marine organisms. However, the current knowledge is limited on how internal nutrient loading affects flora succession when marine ecosystems are restored by flooding nutrient-enriched agricultural land (Nordstrom et al., 2016). The sequence of emerging benthic flora, including microalgal biofilms, macroalgae, and plants, is particularly important for the long-term ecological quality of coastal lagoons restored by MR.
Ecological succession of benthic primary producers within a newly flooded MR area is controlled by the prevailing physical, chemical, and biological factors (Hughes et al., 2009; Mossman et al., 2012; Sullivan et al., 2017; Gerwing et al., 2020). Physical factors include the availability of suitable attachment sites, and the proportion of soft and hard substrata is crucial for the recruitment of flora communities (Raffaelli and Hawkins, 1999; Dale et al., 2019). Macroalgal settlement depends on hard substrata such as stones and shells, whereas seagrasses require soft sediment (Hemminga and Duarte, 2000). Water depth, light penetration, tidal exposure, wave stress, and sediment stability are other physical factors impacting primary producer development (Raffaelli and Hawkins, 1999; Koch, 2001; Kraufvelin, 2007). However, nutrient availability is probably the most important chemical factor that in combination with biological interactions among species determines the succession (Middelboe and Sand-Jensen, 2000; Raven and Taylor, 2002; Thorsen et al., 2019). Accordingly, the dominance among species will be controlled by their specific nutrient affinities and growth rates (Clayton, 1992; Lotze et al., 2001; Tabak et al., 2018), as well as nutrient uptake rates, assimilation efficiencies, and storage capacities (Wallentinus, 1984; Martínez et al., 2012; Kim et al., 2014). Fast growing opportunistic macroalgae are typically short-lived and may dominate when a constant high N and P nutrient supply sustains maximum growth (Pedersen and Borum, 1996; Pedersen et al., 2010; Martínez et al., 2012). The N-fixation ability and rapid growth of mat forming benthic cyanobacteria is important for the ecological succession in N limited and P replete marine environments (Stal, 1995; Bartoli et al., 2018). In contrast, perennial macroalgae grow slower and can store excess nutrients when available, enabling them to maintain growth during periods of low nutrient availability (Pedersen and Borum, 1997; Pedersen et al., 2010; Pedersen and Johnsen, 2017).
Inventories of N and P nutrients in agricultural soils are usually high due to many years of fertilizing crops and/or livestock manure dropping (Rubæk et al., 2013; Sebilo et al., 2013). Flooding by MR inevitable induces an initial rapid release of the stored nutrients from the soils, when PO43– is desorbed following Fe(III) reduction to Fe(II) in the flooded anoxic soil and when NH4+ is desorbed by ion exchange with Na+ from the incoming seawater (Sundby et al., 1992; Rysgaard et al., 1999; Kristensen et al., 2020). The internal nutrient loading and ratio between particularly DIN (= NH4+ + NO2– + NO3–) and DIP (= PO43–) concentration in the water column will most certainly affect flora succession after MR. High nutrient levels typically result in massive blooms of opportunists, such as green macroalgae and benthic cyanobacteria (e.g., Sundbäck et al., 2003; Teichberg et al., 2010; Thorsen et al., 2019). These large blooms can potentially limit species diversity of the associated benthic flora and fauna communities by shading and asphyxiation (Green et al., 2014; Bittick et al., 2018; Valdemarsen et al., 2018). Opportunistic and short-lived species with low internal nutrient storage are often limited by N, but rarely by P in eutrophic waters (Wallentinus, 1984; Pedersen and Borum, 1996; Pedersen et al., 2010). Accordingly, when nutrient pools are exhausted, the opportunistic pioneers will diminish, and slower growing late-successional species can colonize successfully (Valiela, 1984; Raven and Taylor, 2002; Alestra and Schiel, 2014).
The biological interactions among macroalgae and microphytobenthos (i.e., diatoms and cyanobacteria) are therefore primarily controlled by competition for nutrients, light, and surface space (Stal, 2001; Sundbäck et al., 2003; Bolhuis et al., 2013; Fong and Fong, 2018). In addition, grazing by invertebrates (e.g., isopods and amphipods) and birds may affect the outcome of the competitive interaction by hampering the occurrence of palatable opportunistic species (Gagnon et al., 2016; Schagerström and Kautsky, 2016; Marks et al., 2020). However, the exact outcome of biological interactions involving macro- and microbenthic primary producers under various nutrient regimes and environmental settings in MR locations is unpredictable, and new knowledge can only be gained by conducting detailed assays and monitoring of key parameters, such as nutrient loading and algal development.
The aim of this study was to explore the ecological succession of benthic primary producers (macroalgae and benthic cyanobacteria) by detailed assays after the establishment of Gyldensteen Coastal Lagoon in Denmark by MR of reclaimed coastal farmland. The development of primary producers was monitored for 5 years after flooding, and the observed succession was coupled to internal loading and dynamics of nutrients within the lagoon. The newly restored Gyldensteen Coastal Lagoon serves as a case study for understanding the wider ecological effect of MR on primary producer communities by elucidating the successional time frames and nutrient-driven tipping points. Our hypotheses were as follows: (A) The macroalgal community is dominated by opportunistic species the first years after flooding when internal nutrient loading is high; (B) The levels and relative proportion of primarily DIN and DIP are the driver of changes in primary producer communities along the ecological succession; C) Cyanobacteria dominates when other primary producers are limited by N availability.
Materials and Methods
Study Site
The restored Gyldensteen Coastal Lagoon is the first large-scale managed realignment (MR) project in Denmark. It is located along the north coast of the Island Fyn (Figure 1). The area was drained and diked by the Gyldensteen Estate in the 1870s and used for farmland services until Aage V. Jensen Nature Foundation purchased 616 ha of the land in 2011. An area of 214 ha was prepared with new inland dikes before the old seaward dikes were deliberately breached on 29 March 2014 to restore a marine lagoon with an average water depth of about 1 m. The seaward openings allow tidal exchange with an average range of ± 20 cm, while occasionally wind-driven events may lead to changes in water level of more than ± 100 cm. Even mild wave disturbance results in resuspension of silt and clay from the soil of the shallow lagoon. There is no freshwater discharge, except for 1–2 annual winter overflows from a neighboring freshwater wetland east of the lagoon. Salinity is therefore similar to that in the outside water body, Kattegat (20–25). The near-absent catchment area and low freshwater input make Gyldensteen Coastal Lagoon a perfect location for studying how internal nutrient loading from the agricultural past affects ecological succession in newly restored marine areas, where external nutrient sources are negligible.
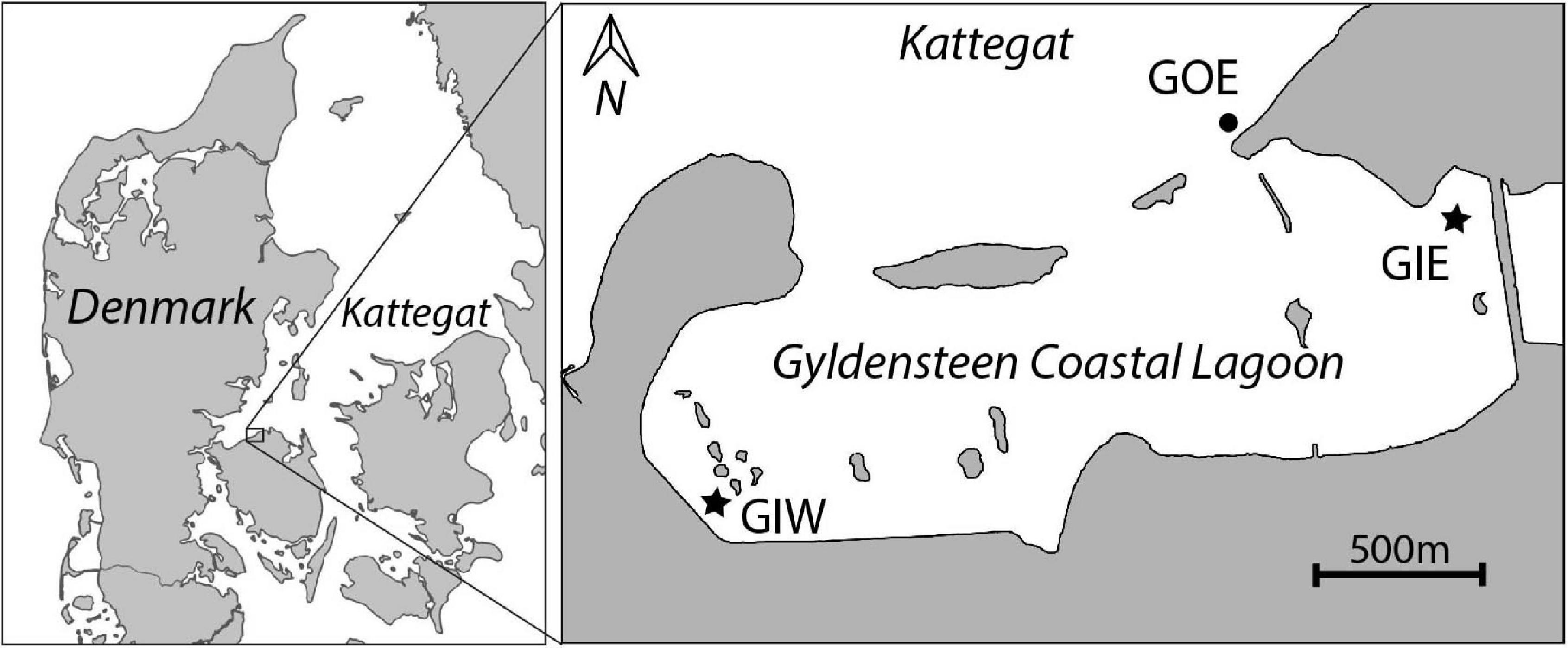
Figure 1. Map of Gyldensteen Coastal Lagoon in Denmark (inserted map) with location of monitoring and sampling stations GIE, GIW, and GOE indicated.
We established two sampling stations inside the lagoon, Gyldensteen Inner Eastern (GIE) and Gyldensteen Inner Western (GIW), while one station outside the eastern entrance, Gyldensteen Outer Eastern (GOE), acted as an undisturbed coastal control (Figure 1). Mapping surveys proved that GIE and GIW are representative for the major bottom types and water depths covering about 70–80% of the lagoon (Kristensen et al., 2020). Station GIE was located in a stubble field from the last grass crops in 2013, while station GIW represented bare soil disturbed by the construction of the new inland dikes in 2013. The dikes near GIE are protected with large stones (15–30 cm), whereas the dikes near GIW are covered with grass. A few boulders (diameter 50–100 cm) are randomly spread throughout the lagoon. Station GOE was located in sandy sediments with gravel and scattered smaller stones at a water depth similar to the stations inside the lagoon.
Monitoring, Sampling and Analysis
The flora succession was monitored by separating primary producers into macroalgae and benthic cyanobacteria. The macroalgae were further grouped into green, brown and red algae. The presence of each type of primary producer was determined by a semi quantitative areal coverage approach with five levels: 0 (not present), 1 (present with 1–25% coverage); 2 (common with 26–50% coverage), 3 (abundant with 51–75% coverage) and 4 (dominant with 76–100% coverage). The areal coverage at each station was estimated from visual inspections of 10–15 random plots of 25 m2 selected within a pre-determined 100 × 100 m area. The observed coverage within the plots was pooled to provide a total estimate for the whole station per visit. The monitoring procedure was repeated by the same person two times every month in the main growing season from April to October and once every month from November to March for a period of 5 years (2014–2018).
Most macroalgae sampled from the selected plots were identified in the field, and those that could not be identified were brought back to the laboratory for closer examination. Identification was done to the lowest possible taxonomic level and all species were categorized into ecological functional groups. Some closely related species with the same ecological function were of practical reasons not separated during monitoring. These include the two brown algal species Ectocarpus and Pilayella with intertwined thalli. Similarly, Ulva intestinalis and Ulva prolifera (both previously assigned to the genus Enteromorpha) were not separated because of the almost complete dominance of U. intestinalis after year 0. Finally, benthic cyanobacteria were not identified to species-level, but were included as one group of benthic primary producers because of their occasional massive areal coverage in the lagoon. All dominating macroalgal species were sampled in the field and stored in Ziploc bags at 4°C for later processing of tissues for elemental NP analysis. Benthic cyanobacteria were not analyzed for N and P because no samples clean of soil contamination could be obtained.
The collected macroalgae (3–4 replicates from each sampling site and occasion) were rinsed for all visual debris and washed in distilled water to remove salts from the surface. The tissues were then dried at 60°C for 24 h and ground into powder using a plant mill (Retsch MM301). About 2 mg of dried tissue were transferred into tin capsules for N analysis on a Thermo Analytical elemental analyzer, Flash EA 2000 Series. P content was determined after combusting the dried tissues at 520°C for 6 h followed by boiling the ash at 120°C in 1 M HCl for 1 h. The extract was analyzed for DIP by colorimetric analysis according to the molybdate blue method with ascorbic acid (Koroleff, 1983).
To supplement the visual monitoring of benthic cyanobacteria, we sampled four soil cores (i.d. of 5 cm) for assessment of microphytobenthos biomass as Chlorophyll a (Chl a) in surface soil during most monitoring occasions. The top 0–5 mm layer was sliced and stored frozen at –20°C. Chl a was extracted from 0.5 g subsamples with 90% ethanol overnight before absorbance was analyzed at 665 and 750 nm with and without addition of one drop of 2 M HCl (Parsons et al., 1984). We converted soil Chl a to mg m–2 using wet densities (g cm–3) of surface soil measured once per year.
Water samples were obtained manually at the three examined stations and GF/F filtered for nutrient analyses once every 1–3 months from 2014 to summer 2016 and 3 times every month after that. Samples for total dissolved nitrogen (TDN) were analyzed on a Shimadzu TOC-L with TNM-L module and DIN (NH4+ + NO2– + NO3–) was analyzed on a Skalar SAN + + Analyzer System according to the manufacturers guidelines. DON (dissolved organic nitrogen) was determined as the difference between TDN and DIN. DIP (PO43–) was analyzed spectrophotometrically as described above. Water temperature was measured continuously with HOBO temperature loggers during the first 3 years (until 2016), but only manually 4 times each year after that. Sun hour data and air temperature in the nearby town of Bogense was achieved from the Danish Meteorological Institute (DMI). Cumulated sun hours were calculated at monthly intervals from March 2014 to December 2018 by summing the total time (resolution of 0.5 min) where the intensity of sun irradiance exceeded 120 Watt m–2.
Data Treatment and Statistics
The species list was divided into the following groups according to the classification of opportunistic species in the technical guidelines for macroalgal monitoring by DCE (Danish Center for Environment and Energy): Green opportunists, brown opportunists, brown annuals, brown perennials, red opportunists, red annuals, and red perennials (Table 1). Although Agarophyton vermiculophylla (previously referred to as Gracilaria vermiculophylla) is listed as invasive and sometimes opportunistic, we consider this species a red perennial since it survives and grows well during Danish winters (Thomsen et al., 2007a). Furthermore, we have included benthic cyanobacteria as a functional group, but excluded these from the calculation of species richness.
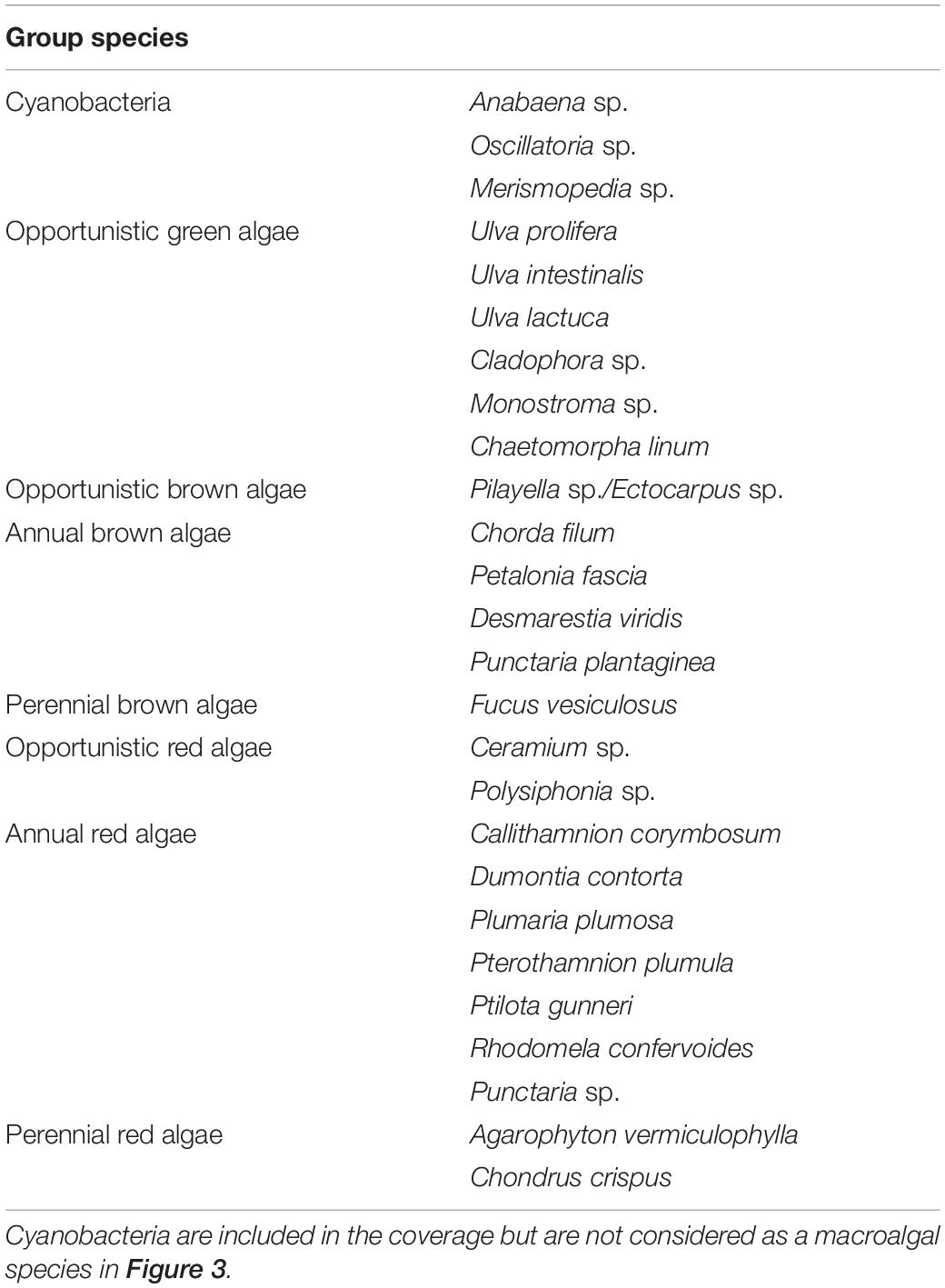
Table 1. List of identified cyanobacterial and macroalgal species divided into opportunistic, annual, and perennial groups.
Bimonthly and monthly macroalgal cover and species richness estimates were correlated (Pearson) with the corresponding water DIN, DIP, and DIN:DIP ratio as well as light to detect any interactions and seasonal effects. Tissue N:P of dominating macroalgae (U. intestinalis, Cladophora sp., F. vesiculosus, Pilayella sp., A. vermicullophylla, and Ceramium sp.) was correlated with water DIN:DIP ratio at both stations. Year-to-year variations (factor a) and differences between stations (factor b) were tested by 2-way ANOVA using average species richness observed during the growth season from April to October. Furthermore, average values of group coverage, species richness, and soil Chl a in the growth season were year to year correlated with corresponding water DIN and DIP. The approach by including only growth season data was applied to determine temporal and spatial changes when growth and species richness were highest.
Results
Temperature and Insolation
Water temperature and light were high during the summer of 2014, reaching a level of 25°C and about 290 cumulated sun hours in July (Figure 2). The following three summers (2015-2017) were colder and slightly less sunny, while the sunny summer months of 2018 reached between 292 and 367 cumulated sun hours. However, the highest water temperature recorded this year only reached 23°C, probably due to the lack of continuous temperature monitoring. Average daily air temperature (at 15 m height) in July provided almost similar levels around 20°C in 2014 and 2018, which was 2–3°C higher than in 2015, 2016, and 2017.
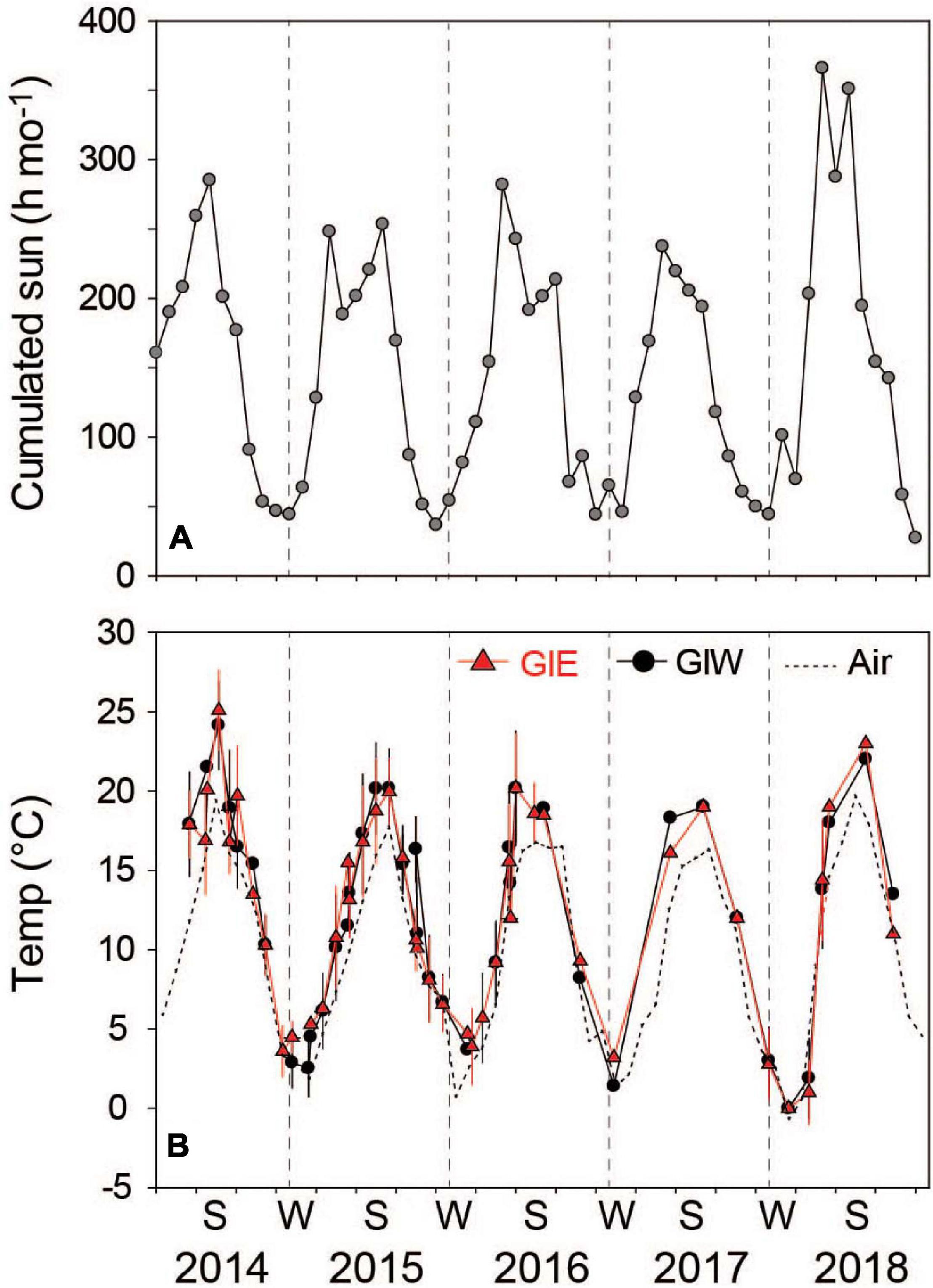
Figure 2. (A) Cumulated sun hours in the nearby town of Bogense at monthly intervals from March 2014 to December 2018 (from Danish Meteorological Institute, DMI). (B) Monthly average water temperature in Gyldensteen Coastal Lagoon obtained from HOBO temperature loggers from June 2014 to August 2016 and manually 4 times each year after that. Dotted line indicates air temperature in the nearby town of Bogense achieved from DMI. Lines and symbols in red represent station GIE and in black represent station GIW.
Species Richness and Succession of Vegetation Cover
There was a total of 22 macroalgal genera with at least 24 species registered at the two monitoring stations in Gyldensteen Coastal lagoon during the first 5 years after flooding (Table 1), although not all were present at the same time and location. Species richness showed strong seasonality for green, brown and red macroalgae in all the examined years with 1–5 species during winters and 4–10 species during the growth seasons (Figure 3). The slightly higher species richness at GIE than GIW was not significant (p > 0.05). The average growth season species richness at both stations increased significantly (p < 0.001) from 2.8 ± 1.4 species in 2014 to 6.7 ± 1.2 species in 2015 and after a decline to 5.7 ± 1.7 species in 2016 and 4.6 ± 1.5 species in 2017, it increased again to 6.7 ± 1.8 species in 2018 (p < 0.05). All macroalgal species present within the first year; the green algae Cladophora sp., U. intestinalis and U. prolifera; the brown algae Pilayella sp./Ectocarpus sp.; and the red alga Ceramium sp., are classified as opportunistic species in Danish waters (Høgslund et al., 2014). Only U. intestinalis (present from June 2014), the brown alga F. vesiculosus (present from October 2014) and the red alga A. vermiculophylla (present from May 2015) were observed at one or both station during almost every visit after their first occurrence. The opportunists generally flourished in summer and disappeared during winter.
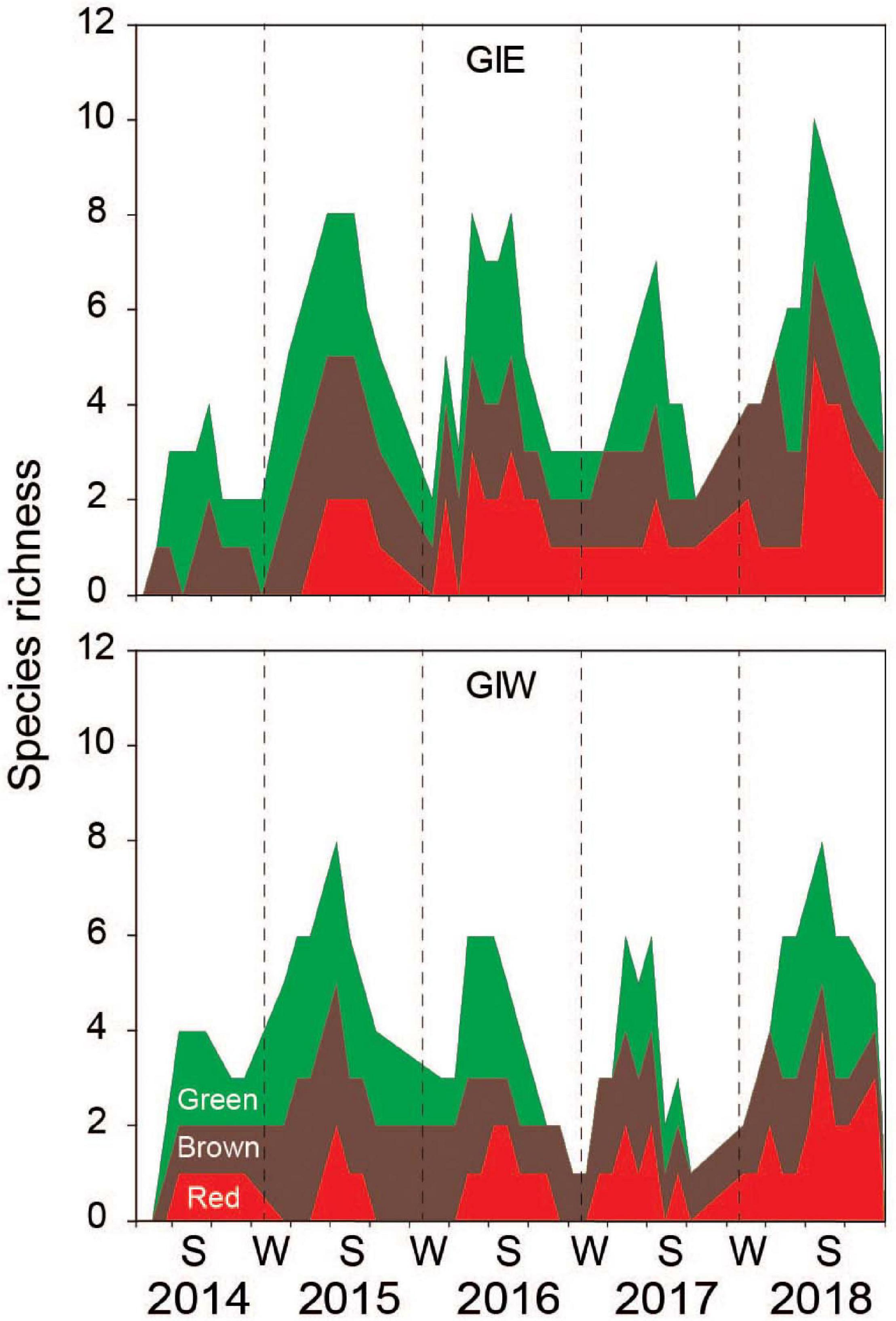
Figure 3. Species richness of macroalgae at station GIE and GIW in Gyldensteen Coastal Lagoon from April 2014 to December 2018. The data are given as cumulated red + brown + green macroalgal species at each date as indicated by the corresponding color codes.
Succession of flora cover was initiated by a short-term bloom of benthic cyanobacteria in May 2014 covering more than half of every available surface in both east (GIE) and west (GIW) of the lagoon (Figure 4). Cyanobacteria were in June 2014 quickly replaced by a dense cover of green algae, particularly at GIE. The coverage of cyanobacteria remained low at both stations in 2015 and 2016 until a small secondary benthic cyanobacterial burst appeared in September 2016 at GIW. This bloom continued in the summer of 2017 where it reached 100% cover at both stations (Supplementary Figure S1). After a decline during winter, cyanobacteria returned in late summer of 2018, but only with 51–75% areal cover. In accordance with this pattern, Chl a in surface soil was generally high in early summer of 2014 with levels ranging from 122 to 342 mg m–2 at GIE and from 118 to 211 mg m–2 at GIW (Figure 5). Subsequently, Chl a decreased to more modest background values around 100 mg m–2 in 2015 and 2016 until a sudden peak to 482 mg m–2 occurred at GIW in September 2016. Soil Chl a returned in 2017 to the high 2014 levels before decreasing to levels around 50 mg m–2 in early 2018, followed by peaks of 250–300 mg m–2 during early fall this year. Soil surface Chl a content correlated significantly with the cyanobacterial cover at both GIE (p = 0.003, r2 = 0.40) and GIW (p = 0.016, r2 = 0.23) during the growth season (April–October) of all years.
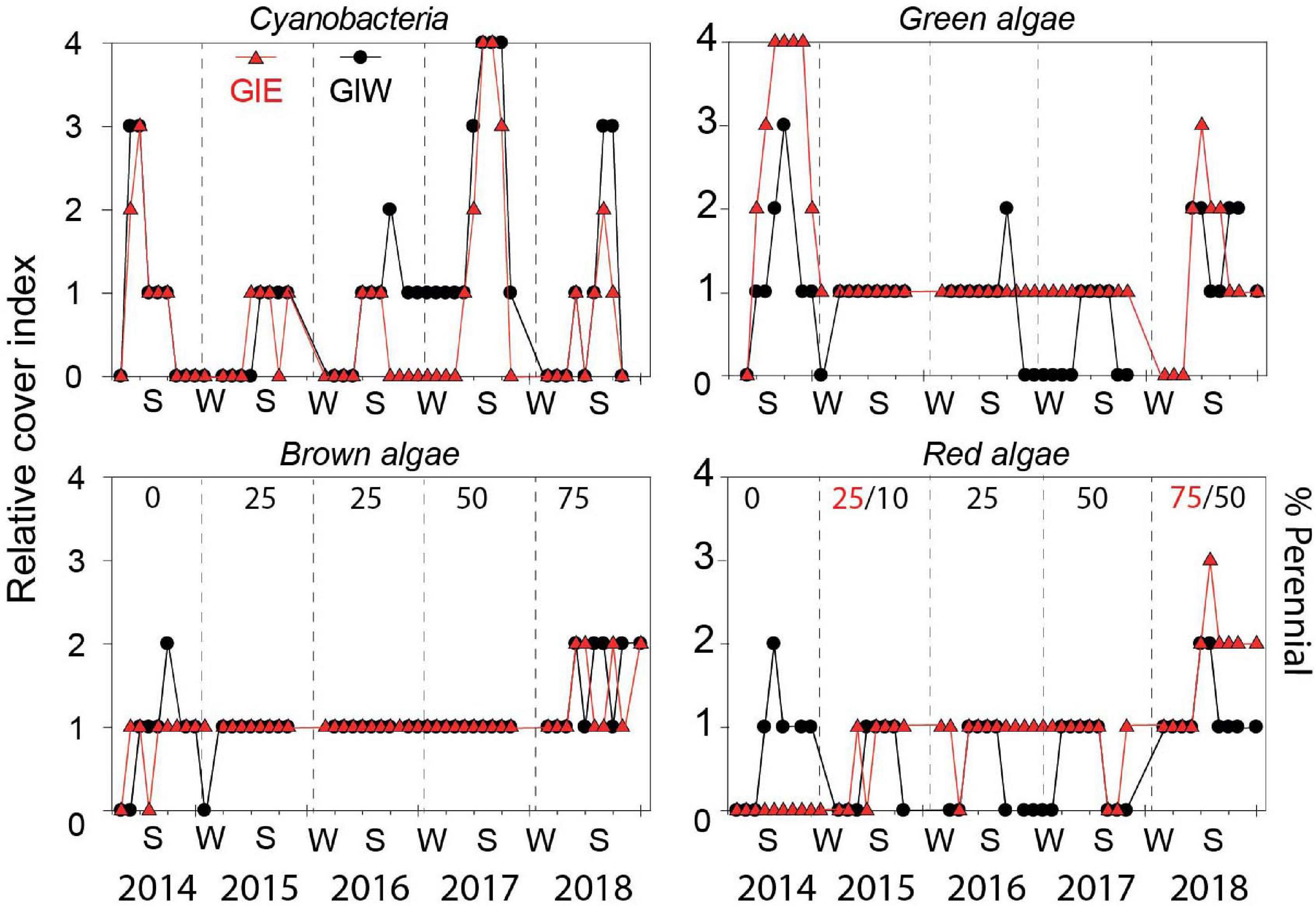
Figure 4. Relative cover of cyanobacteria, green algae, brown algae, and red algae in Gyldensteen Coastal Lagoon from April 2014 to December 2018. The relative cover index is given as 0 (not present), 1 (present with 1–25% coverage), 2 (common with 26–50% coverage), 3 (abundant with 51–75% coverage), and 4 (dominant with 76–100% coverage). The percentage cover by perennial species is shown by numbers in black (red indicate GIE and black GIW only when stations differ) for brown and red algae. Lines and symbols given in red represent station GIE and in black represent station GIW.
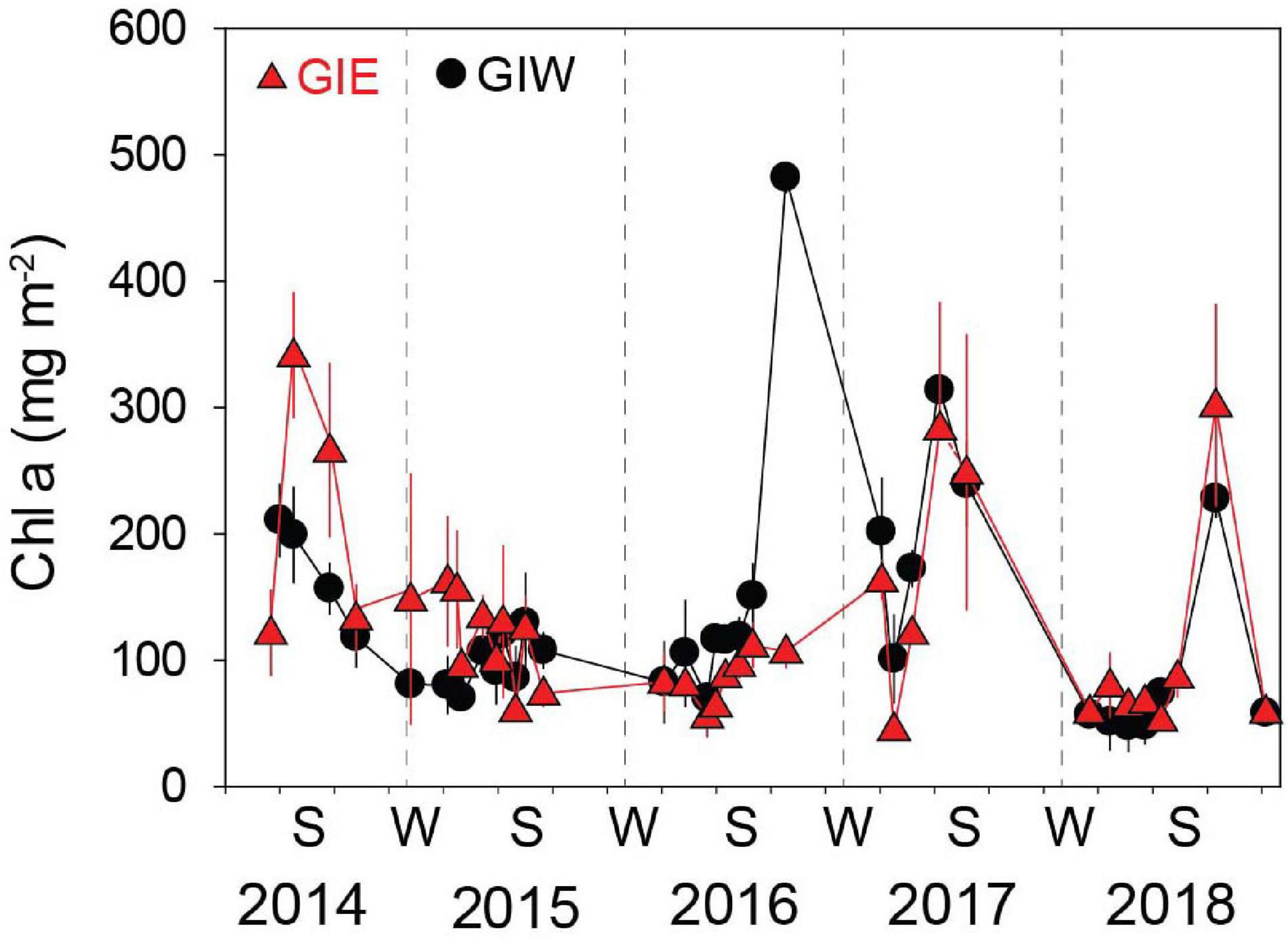
Figure 5. Chlorophyll a (Chl a) in the 0–5 mm surface soil at Gyldensteen Coastal Lagoon from April 2014 to December 2018. Lines and symbols in red represent station GIE and in black represent station GIW. Results are given as average ± se (n = 3).
The massive bloom of opportunistic green macroalgae (Figure 4) in 2014 with 100% cover from July to October formed up to 30 cm thick mats at GIE (Supplementary Figure S1). This bloom was dominated by Ulva intestinalis (and partly U. prolifera) in July and shifted to Cladophora sp. in August-October. The cover at GIW only reached up to 75% and the mats were thinner than at GIE. Partly degraded Cladophora sp. was still present over the following winter, but the remaining thalli observed in the spring failed to bloom during summer 2015. In this and the following 2 years (2016–2017), green macroalgae only covered < 25% but with the presence of scattered tube forming Ulva (U. intestinalis and U. prolifera) and to less extent leaf-forming U. lactuca and Monostroma sp. attached to large stones and stubble remains from the agricultural past. In the warm and sunny summer of 2018, Cladophora sp. coverage increased again to 51-75%, but without reaching the first-year areal extent and mat thickness.
Brown algae were dominated by few species of Ectocarpus sp./Pilayella sp. (denoted opportunistic brown) generally with < 25% coverage in 2014. Coverage of these opportunistic brown algae was higher at GIW than GIE during the summer although rarely reaching 26–50% (Figure 4). A few juvenile thalli (1–2 cm) of the perennial brown alga Fucus vesiculosus were in October 2014 discovered attached to stones along dikes. The following 3 years (2015-2017), brown algal cover was <25% (Figure 4) with few opportunistic species appearing in spring and declining during autumn (Figure 3). In contrast, the proportion of F. vesiculosus coverage increased steadily at both stations from 2015 to 2018 (Figure 4), and the distinct increase in total brown algal cover to 26–50% in 2018 was solely due to growth of this species.
A similar shift from opportunistic to perennial species occurred for red algae during the 5-year study period. The opportunists Ceramium sp. and Polysiphonia sp. were generally restricted to GIW with coverages of < 25% in 2014 and only more than 25% in August (Figure 4). The perennial A. vermiculophylla was first registered in May 2015 and expanded quickly in the lagoon and became the dominating red algal species in 2017 and 2018. The coverage of A. vermiculophylla reached 51–75% at GIE in summer 2018 (Figure 4), while other red algae remained rare (<25%) despite the increasing species richness this year (Figure 3).
Nutrient Relationships
Water column DIN showed strong temporal variations with low concentrations during the growth season and high concentrations in the dark winter months (Figure 6). The winter peaks reached concentrations of 52.4 ± 0.5 (GIE) and 54.6 ± 0.5 μM (GIW) in the first winter (2014/2015), which was 3 times higher than at GOE outside (16.7 ± 7.2 μM). Winter peak concentrations inside the lagoon steadily decreased the following years and reached in 2017/2018 only ∼25% of the 2014/2015 level. The growth season DIN concentrations were low in all years and varied from 0.2 to 7.2 μM at GIE and 0.4 to 6.8 μM at GIW. DIN concentrations inside the lagoon reached levels both summer (<3 μM) and winter (15–20 μM) in 2017–2018 that were similar to or even lower that outside (station GOE). The seasonal variation in water column DON at GIE showed high summer and low winter levels (Figure 7). DON rarely declined below 20 μM during winter and reached levels exceeding 60 μM during the green algal blooms in the summer of 2014 and in the warm summer of 2018. The increasing DON concentrations in 2017 and 2018 occurred in associated with the bloom of benthic cyanobacteria. DON was in general 5–15 μM lower at GOE (and GIW) than at GIE and followed the same overall temporal pattern, except for ∼30 μM difference in summer 2014 and winter/spring 2018. Water column DIP concentrations were always highest during late summer and lowest in early spring (Figure 6). Summer (June-August) concentrations just after flooding in 2014 were 3 (GIE) and 5 (GIW) times higher inside the lagoon (GIE: 2.8 ± 1.0 μM and GIW: 4.9 ± 1.5 μM) than outside (GOE: 1.0 ± 0.1 μM). The summer maximum inside the lagoon decreased to about half the initial level the following years (2015-2018), while the level outside remained almost constant over time.
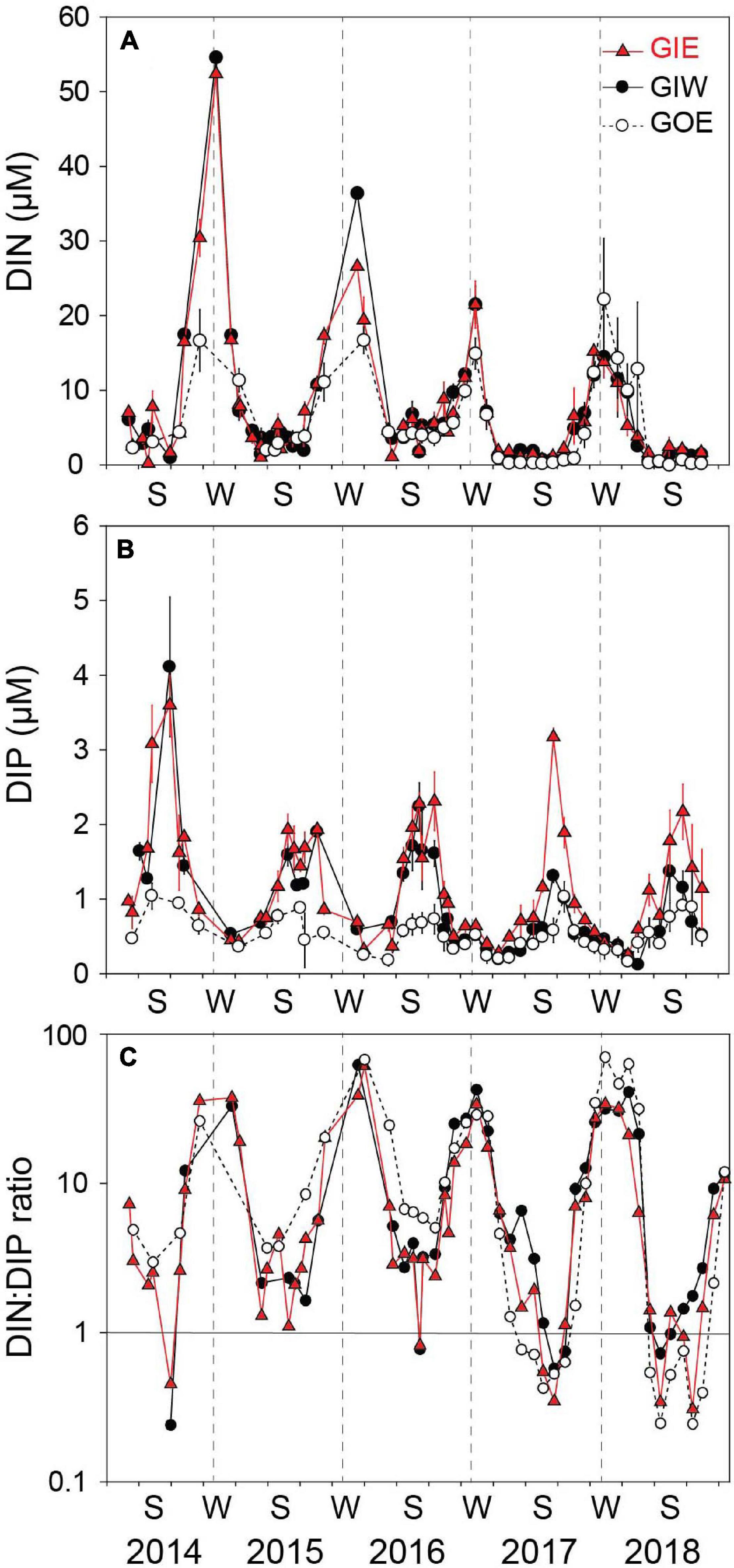
Figure 6. (A) DIN concentration, (B) DIP concentration, and (C) DIN:DIP ratio in the overlying water at station GIE, GIW, and GOE in Gyldensteen Coastal Lagoon from April 2014 to December 2018. Values are given as average ± se of triplicates sampled once every 1–3 months from April 2014 to June 2016 and triplicate monthly samples every month after that.
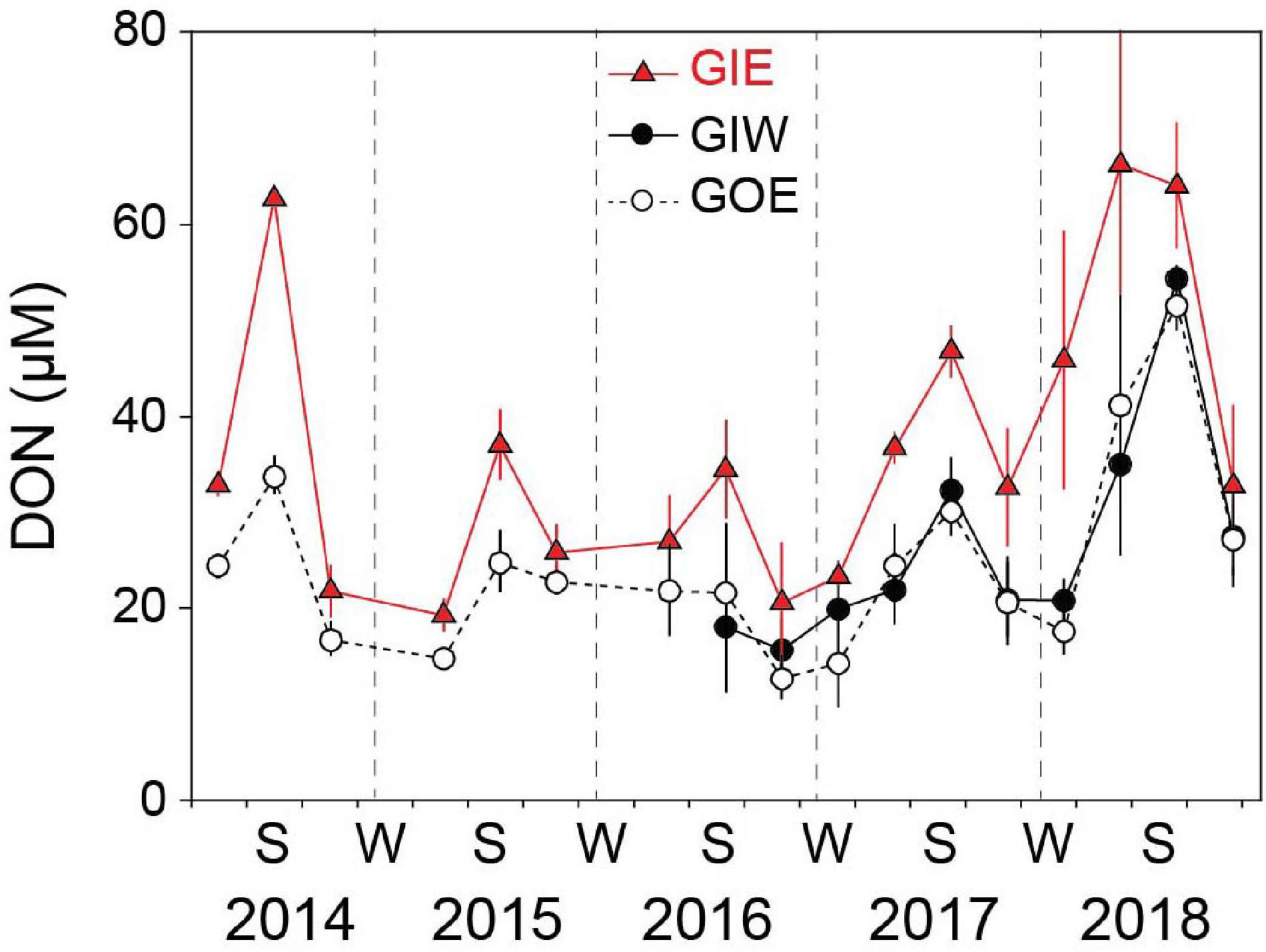
Figure 7. Seasonal DON variations in the overlying water at station GIE, GIW, and GOE in Gyldensteen Coastal Lagoon from 2014 to 2018. Values are given as average ± se of samples taken once every 1–3 months from 2014 to spring 2016 (n = 3) and triplicate monthly samples every month after that (n = 9).
Water column DIN:DIP molar ratios largely followed the seasonality of DIN and DIP (Figure 6) with positive linear correlation between DIN and DIN:DIP ratio (GIE: p < 0.001, r2 = 0.71 and GIW: p < 0.001, r2 = 0.77) and negative logarithmic correlation between DIP and DIN:DIP ratio (GIE: p < 0.05, r2 = 0.34 GIW: p < 0.05, r2 = 0.16). Summer (June-August) DIN:DIP ratios were at both stations low and with few exceptions almost similar around 2–3 from 2014 to 2016 but decreased below 1 in summer 2017 and 2018 (Figure 6). Highest winter (December–February) DIN:DIP ratios at GIE and GIW ranged from about 30 to 60 throughout all years. DIN:DIP ratios outside the lagoon (GOE) were similar to those for the stations inside, except for a somewhat higher level in summer 2015 and 2016, and winter 2017/2018.
The growth season cover of both green algae and benthic cyanobacteria was positively correlated with DIP at GIE (p < 0.05, r2 = 0.22 and 0.24, respectively), while brown algal cover was negatively related to DIN and both GIW and GIE (p < 0.05, r2 = 0.21 and 0.42, respectively). Accordingly, cyanobacterial cover was negatively correlated with DIN:DIP ratio at GIW and GIE (p < 0.05, r2 = 0.23 and 0.35, respectively). However, green algal cover was only correlated with DIN:DIP ratio at GIE (p < 0.05, r2 = 0.23). No other relationships were evident between inorganic nutrients and macroalgal abundance or cover, indicating the importance of other seasonal factors (e.g., DON) for growth and species richness.
Tissue N:P molar ratios of the dominant algal species varied seasonally according to DIN and DIP availability. The seasonal pattern of tissue N:P was most pronounced and varied similarly at GIE and GIW for the perennial F. vesiculosus and A. vermiculophylla (Figure 8). Low DIN and high DIP levels during summer resulted in low tissue N:P ratios, while surplus of DIN during winter generated high N:P ratios in the overwintering perennials. Highest tissue N:P > 30 was generally observed in April and lowest values of 10-20 occurred in August. Tissue N:P values of F. vesiculosus were consistently displaced about 10 units lower than those of A. vermuculophylla. N:P ratios in the dominant green algae, Cladophora sp. and U. intestinalis, were similar at levels mostly ranging from 15 to 30 (Figure 8). A weak seasonal variation was evident for U. intestinalis at GIE with a tendency for lower N:P ratios in summer than spring, except for summer in 2018 where the N:P ratio increased drastically to more than 40. Cladophora sp. tissue N:P ratios showed no clear seasonal trend. The temporal variation of tissue N:P in these opportunistic green algae was masked because they only were recovered during the growth season.
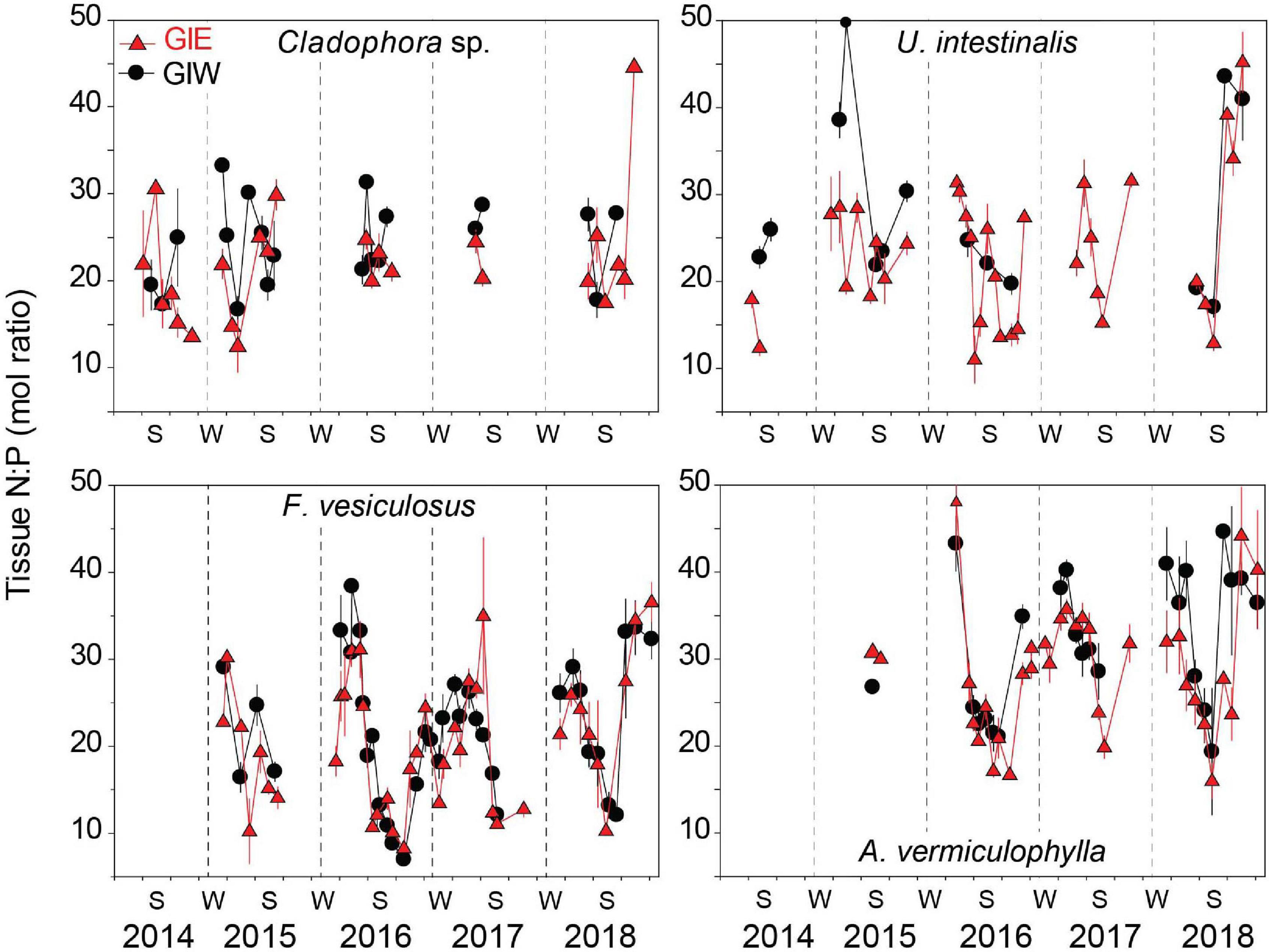
Figure 8. Tissue N:P molar ratio of the common macroalgae, Cladophora sp., U. intestinalis, F. vesiculosus, and A. vermiculophylla, in Gyldensteen Coastal Lagoon. Lines and symbols in red represent station GIE and in black represent station GIW. Samples of all species were not available at every sampling date from April 2014 to December 2018 as indicated by the gaps in the data series. Values are given as average ± se (n = 3).
Discussion
This study demonstrates that the first 5 years of macrophyte succession in the restored Gyldensteen Coastal Lagoon after MR of agricultural land were primarily controlled by nutrient availability. The water column nutrient concentration was at any time a balance between internal nutrient loading from the flooded soil and loss by algal assimilation as well as rapid tidal water exchange (residence time of 2–3 days) (Kristensen et al., 2020). Based on a stable isotope approach, Thorsen et al. (2019) proved the importance of fertilizer-derived nutrients from the soil for macroalgal growth in the same lagoon. Since the N pools in the soil appeared labile and was rapidly exhausted, DIN concentrations in the water column inside Gyldensteen Coastal Lagoon decreased considerably during the 5 years to a level similar to or even lower than outside the lagoon. DIP concentrations, on the other hand, remained 2-3 times higher than outside and only decreased slowly due to steady supplies from large but relatively slow reacting P pools in the flooded agricultural soil (Kristensen et al., 2020). This differentiated temporal change in internal N and P supply gradually shifted the algal dominance from opportunistic annual species toward perennial species with intermediate bursts of benthic cyanobacterial cover. Accordingly, the 5 year algal succession in Gyldensteen Coastal Lagoon had the following four major phases (Supplementary Figure S1): (1) A massive green algal bloom driven by excessive internal nutrient loading right after flooding (2014); (2) Reduced algal cover and increasing species diversity caused by gradually diminished DIN loading (2015–2016); (3) Development of a benthic cyanobacterial bloom stimulated by continued DIP loading when DIN limitation was strongest (2017); and (4) Higher growth and diversity of all algal groups driven by stimulated mineralization and nutrient loading in a warm and sunny year (2018). It should be noted, though, that herbivores (e.g., amphipods and isopods) may influence the outcome of competition among marine macrophytes through preferential consumption of the most palatable species (Van Alstyne et al., 1999; Alestra and Schiel, 2014; Gagnon et al., 2016). Grazing impact is not emphasized in the present study, but we are confident that nutrient availability is of primary importance for the outcome of competition in Gyldensteen Coastal Lagoon due to a general scarcity of herbivores (pers. obs.).
Initial Phase With Blooms of Benthic Cyanobacteria and Opportunistic Green Algae
The pioneer species that initiated the succession at Gyldensteen Coastal Lagoon were supported by the excessive loading of DIN and DIP from the soil immediately after restoration. The brief bloom of N-fixing benthic cyanobacteria that was observed in May 2014 before the green algae proliferated indicates unstable conditions and higher DIP than DIN availability probably due to fast release of DIP and “delayed” DIN release from the flooded soil (Figure 4). PO43– is released instantly by desorption when the soil turns anoxic and Fe(III) is reduced to Fe(II) right after flooding (Matthiesen et al., 2001; Kraal et al., 2015; Kristensen et al., 2020), while NH4+ is released by cation exchange on particle surfaces when excess Na+ from the saline water gradually diffuses into the soil (Gardner et al., 1991; Rysgaard et al., 1999). The first field measurements of soil-water nutrient fluxes in June 2014, 2–3 months after flooding, showed a substantial release of both DIN and DIP (Kristensen et al., 2020). However, a simultaneous laboratory study revealed that DIN (mostly NH4+) efflux first peaked 20–30 days after flooding of Gyldensteen soils with seawater (Sjøgaard et al., 2018). The delay in DIN loading may have triggered cyanobacterial growth in the DIP replete environment, allowing a brief bloom that colonized all surfaces in May 2014.
The bloom of benthic cyanobacteria ceased in June 2014 and was followed by a massive green algal bloom (Figure 4). The opportunistic green algae responded quickly to the increased (but delayed) loading of DIN from the soil during early summer and was augmented by optimal insolation (Thorsen et al., 2019; Kristensen et al., 2020). Green algae may under such conditions reach growth rates of 0.25-0.53 d–1 (Pedersen and Borum, 1997), which is far beyond cyanobacterial growth rates of 0.11-0.17 d–1 (Marino et al., 2003; Stuart et al., 2016). Thus, Björnsäter and Wheeler (1990) reported that tissue N:P ratios of U. intestinalis and U. fenestrate between 16 and 24 indicate ample supply of both DIN and DIP, which is consistent with the ratios observed for Cladophora sp. (17–30) and U. intestinalis (12–25) in summer 2014 at Gyldensteen Coastal Lagoon (Figure 8). The benthic cyanobacteria were probably overgrown and shaded by the bloom of rapid growing green macroalgae through competition for light and nutrients (Fong et al., 1993a, b; Howarth and Marino, 2006), as observed at station GIE, but less so at GIW due to a more scattered green algal cover (Figure 4). Since cyanobacterial growth is controlled by a complex interaction of physical, chemical, and biological factors (Pinckney et al., 1995; Thacker and Paul, 2001; Howarth and Marino, 2006), other causes may be involved in the simultaneous lagoon-wide summer decline in benthic cyanobacteria. The less sheltered conditions at GIW and faster water exchange with the outside may lead to stronger loss of cyanobacteria at this location due to lowered DIP concentrations and high physical stress (Thacker and Paul, 2001). The green algal bloom, that was potentially DIN limited during summer 2014, may have been supplemented by high levels of DON (Figure 7) delivered from the algal bloom itself (Tyler et al., 2001; Maie et al., 2006). DON has the potential as a source to macroalgae with 10 to 25% bioavailable N (Guldberg et al., 2002; Maie et al., 2006), either through direct uptake (e.g., urea and amino acids) or indirectly after fast remineralization to DIN (Van Engeland et al., 2013; Shpigel et al., 2019).
The presence of opportunistic brown and red algae was limited the first year in Gyldensteen Coastal Lagoon by competition for space and nutrients, especially at GIE where the total green algal cover prevented any other colonization (Figure 4). Brown (Pilayella/Ectocarpus) and red (Ceramium sp.) opportunists are relatively fast growing under high nutrient supply, but their propagules were probably inhibited by competition for nutrients and light from the massive green macroalgal cover (Middelboe and Sand-Jensen, 2000; Korpinen et al., 2007; Kraufvelin, 2007).
The Intermediate Phase With Increasing Diversity
The low coverage of all algal groups in 2015 and 2016 was unexpected and partly invalidated our hypothesis that predicted long lasting dominance of opportunistic algae (Figure 4). The rapid decrease in algal cover was apparently coincident with a steep decline in internal nutrient loading from 2014 to 2017 (Kristensen et al., 2020). The high N availability in 2014 was only evident as significantly higher summer DON, but not DIN concentrations in the water compared with 2015 and 2016 (Figure 6) due to rapid and concurrent uptake of particularly DIN by the massive green algal bloom (Valiela et al., 1997; Kraufvelin, 2007; Thorsen et al., 2019). In contrast, summer DIP levels were in all years above the required concentrations for the opportunistic species present in the lagoon (Wallentinus, 1984; Pedersen and Borum, 1996; Pedersen et al., 2010). During spring, tissue N:P ratios > 25 of green algae indicated a potential P limitation before DIP concentrations increased in summer (Figure 8). The low algal coverage in 2015 and 2016 is therefore likely caused by DIP limitation in spring, DIN limitation in summer combined with relatively low irradiance and temperature during the growth season of these years (Figure 2). It was expected that the DIP replete summer conditions would promote benthic cyanobacterial blooms every year, but this was first triggered by the very low DIN levels (<2 μM) and high temperatures in the summer of 2017. Thus, the N supply in 2015 and 2016 may to some extent have been supplemented by DON with higher bioavailability than in 2017.
The increase in species richness at Gyldensteen Coastal Lagoon from 2014 to 2016 (Figure 3) followed the basic principles of succession where pioneering species are gradually replaced by a variety of later successional (e.g., perennial) species when nutrients are diminished (Sousa, 1979; Valiela, 1984; Kraufvelin et al., 2007). However, not all late arriving species with high nutrient affinity sustained stable populations despite ample space for expansion these years. For example, the annual Chorda filum was abundant in 2015, but completely absent in 2016. Since Chorda filum cannot develop in the intertidal zone and prefers the low to upper subtidal (South and Burrows, 1967), extended periods of low water levels during the germination period in spring 2016 may have prevented its establishment. The perennial F. vesiculosus, that is more tolerant to desiccation, gradually became more abundant in 2015 and 2016. It is well adapted to low nutrient availability and can store N and P to sustain growth during limiting periods (Pedersen and Borum, 1996, 1997; Pedersen et al., 2010). The invasive red algae A. vermiculophylla (Thomsen et al., 2007a, b) also colonized the lagoon in 2015 and 2016. This perennial species survived well during winter in the sheltered lagoon due to its tolerance toward extreme temperatures, desiccation and nutrient stress (Nyberg et al., 2009; Nejrup et al., 2013; Pedersen and Johnsen, 2017). The capacity of A. vermiculophylla for fast growth, fast uptake and internal storage of nutrients during spring and summer assured its success in the lagoon (Pedersen and Johnsen, 2017).
Late Succession Phases With Dominance of Benthic Cyanobacteria and Macroalgae
Cyanobacterial mats often dominate shallow marine systems during summer, particularly when macroalgae are DIN limited (Fong et al., 1993b; Littler et al., 2010; Carreiro-Silva et al., 2012). Such conditions apparently prevailed in summer 2017 where a cyanobacterial bloom apparently was driven by high DIP and very low DIN levels (Figure 6). When the mats reached maximum cover (July–September), their benthic filter effect probably prevented further DIN efflux from the soil, leading to even lower DIN loading to the overlying water (Ladakis et al., 2006; Kristensen et al., 2020). Furthermore, it is known that over-consolidated (compacted or compressed) flooded agricultural soils may promote the formation of cyanobacterial mats and hinder ecosystem recovery (Gerwing et al., 2020). Accordingly, the massive cyanobacterial bloom in summer 2017 coincided with a decline in species richness of all macroalgal groups (Figure 3). The cyanobacterial mats probably prevented recruitment, settling, and succession of macroalgae by space competition and prolonged DIN limitation despite a potentially increased N availability in the form of DON (e.g., Williams et al., 2000; Thacker and Paul, 2001).
The apparently ideal conditions for benthic cyanobacteria in 2018 with high DIP, relatively low DIN and an exceptional warm and sunny summer was probably obstructed by higher N availability in the form of DON fed through degradation of the cyanobacterial mats from 2017. All other macroalgal groups including green macroalgae unexpectedly flourished at the expense of cyanobacteria (Figure 4). We propose that cyanobacteria were hampered early in the growth season of 2018 by rapidly growing opportunistic macroalgae stimulated by a relatively high internal DIN and DON loading. DIN levels actually were higher throughout 2018 than in 2017 with July–August concentrations of 1.3–2.4 μM in 2018 compared with 0.6–1.1 μM in 2017, while DON reached the record high levels of 64–66 μM in spring/summer 2018 (Figures 6, 7). This change was probably driven by faster microbial mineralization of cyanobacterial remains and DON/DIN release from the warmer soils in 2018 (Kristensen et al., 2020). The very high tissue N:P (>40) of Cladophora sp. and U. intestinalis in late summer and fall of 2018 (Figure 8) substantiates the ample N supply. Although coexistence of opportunistic macroalgae and benthic cyanobacteria has been recorded (Fong and Zedler, 1993; Fong et al., 1993a, b; García-Robledo and Corzo, 2011), competition for nutrients, space and light between these groups typically regulate their abundance, often leading to exclusion of one or the other (Fong et al., 1993a, b).
We therefore suggest a nutrient dependent tipping point in the balance between macroalgal and benthic cyanobacterial dominance at Gyldensteen Coastal Lagoon. Benthic cyanobacteria with N-fixing capability are favored when DIN:DIP ratios in the overlying water are low (e.g., < 1) and DIN concentrations are below 2 μM (Figure 6). Thus, the higher internal DON/DIN loading in spring and summer 2018 was sufficient to tip the balance in favor of the macroalgae despite the very low summer DIN:DIP ratios. Fong et al. (1993b) similarly concluded that cyanobacteria mats are unable to compete with macroalgae as long as N supply is sufficiently high and only tend to dominate when competition from macroalgae is decreasing late in summer – exactly as observed at Gyldensteen Coastal Lagoon in 2018. The establishment of benthic cyanobacterial mats is not only controlled by nutrient availability. Erosion by physical disturbance (wind and turbulence) may also regulate mat development (Thacker and Paul, 2001; Ladakis et al., 2006). Thus, formation of cyanobacterial mats thick enough to impact living conditions of other benthic organisms require extended calm periods as observed in fall of 2016 and summer of 2017 (Paterson and Hagerthey, 2001; Rossi and De Philippis, 2015). Frequent turbulence and resuspension events at the study site (pers obs) may have suppressed cyanobacteria during periods of otherwise good growth conditions in 2015 and 2018 when there was low cover of opportunistic macroalgae.
The Balance Between Opportunistic and Perennial Macroalgae Is Indicative of Ecological Status
Cladophora sp. has the capacity to outgrow most species of Ulva at high light and temperature conditions during summer when the water DIN:DIP ratio is relatively low (Taylor et al., 2001; Gladyshev and Gubelit, 2019). This is probably the cause for a shift to Cladophora sp. dominance in the eutrophic Gyldensteen Coastal Lagoon during summer 2014. Nevertheless, U. intestinalis could sustain a low and stable coverage under the less nutrient-rich conditions during the following years, probably due to reduced competition for space with Cladophora sp. and supply of N from DON. Opportunistic brown algae rarely bloomed at Gyldensteen Coastal Lagoon despite their ability to respond fast to nutrient enrichments (e.g., Lotze and Schramm, 2000). This opportunistic group was apparently present in the lagoon primarily during the cool spring periods and was outcompeted or nutrient limited in the warmer seasons (Kraufvelin et al., 2007). The gradual change in macroalgal community structure from opportunistic green algal toward perennial brown and red algal dominance in Gyldensteen Coastal Lagoon during the 5-year study period was strongly controlled by the declining internal nutrient loading, particularly DIN availability. Similar nutrient-dependent recovery of perennial macroalgal communities has in recent years been observed in a variety of coastal environments (Pinedo et al., 2013; Rasmussen et al., 2015; Le Fur et al., 2019; Derolez et al., 2020). The moderate DON-based recovery of opportunistic green algae in early summer of 2018 (Figure 4) was apparently not strong enough to preclude substantial growth of the perennial brown (F. vesiculosus) and red algae (A. vermiculophylla). Perennial algae with the ability to store nutrients were, in contrast to the opportunistic green algae, unaffected by declining DIN levels during late summer (Pedersen and Borum, 1997; Pedersen et al., 2010; Pedersen and Johnsen, 2017). Accordingly, tissue N:P ratios of the perennial species reflected the seasonal DIN:DIP variations, but with about two months delay (Figure 8).
Fucus vesiculosus was common on boulders and as drifting clusters at Gyldensteen Coastal Lagoon in 2018, while A. vermiculophylla was attached to small stones on the bare soil throughout the lagoon. Dominance of A. vermiculophylla on bare bottom is a common phenomenon in Danish coastal waters (Nejrup and Pedersen, 2012), while F. vesiculosus typically depends on hard substratum for attachment (Malm et al., 2003). Drifting clusters of F. vesiculosus has been observed frequently along Danish coasts (Valdemarsen et al., 2010; Canal-Vergés et al., 2014), and the drifting form without holdfast and typical air vesicles at Gyldensteen Coastal Lagoon is similar to that reported from the Wadden Sea (Albrecht, 1998). Both F. vesiculosus and A. vermiculophylla are associated with nutrient poor environments (Wikström et al., 2016), while fast growing opportunistic green and brown species are indicative of eutrophic conditions (McGovern et al., 2019). The gradual shift from opportunistic to perennial macroalgal species in Gyldensteen Coastal Lagoon therefore conforms with recognized ecological succession patterns (Sousa, 1979; Valiela, 1984), and implies that this newly flooded environment progresses toward an improved ecological status within a period of 5 years (Wells et al., 2007). This process can, however, be obstructed by benthic cyanobacterial blooms if low DIN levels are not associated with a concurrent lowering of DIP loading from the flooded soil.
Conclusions
The restored Gyldensteen Coastal Lagoon is a rare example of MR where agricultural land is flooded with seawater. We show that opportunistic macroalgae respond rapidly to high initial nutrient loading to the water column from the newly flooded agricultural soils. We also provide evidence for the potential importance of nutrient dynamics involving DON for the macroalgal succession. MR in Gyldensteen Coastal Lagoon benefited from a rapid water exchange and low external nutrient loading from the surrounding watershed and combined with rapidly decreasing internal loading, DIN concentrations reached a level within 4–5 years similar to that in the outside ocean. The decline in DIN limited the growth of opportunistic species, while sustained DON availability resulted in higher species richness and dominance of perennial species, implying that Gyldensteen Coastal Lagoon progresses toward an improved ecological status. However, the persistently high internal loading of DIP maintains the lagoon at an unstable tipping point where small variations in DIN levels, around 2 μM, rapidly can shift the balance between macroalgal and benthic cyanobacterial dominance. It is therefore imperative for future MR projects involving agricultural land to prepare the soil in advance to prevent sustained internal nutrient loading by, e.g., prohibited fertilization several years before flooding. Particularly P fertilization should be avoided to minimize the chances for recurrent cyanobacterial blooms.
Data Availability Statement
The original contributions presented in the study are included in the article/Supplementary Material, further inquiries can be directed to the corresponding author/s.
Author Contributions
SWT planned and conducted the work in collaboration with the other coauthors and wrote the first draft of the manuscript. MH participated in the planning of the work and commented on the draft manuscript. COQ and TV participated in the planning and execution of field work and commented on the draft manuscript. EK participated in the planning and execution of field work, commented on the early drafts of the manuscript, and finalized the writing process. All authors contributed to the article and approved the submitted version.
Funding
Funding for this research was provided by the Aage V. Jensen Nature Foundation.
Conflict of Interest
The authors declare that the research was conducted in the absence of any commercial or financial relationships that could be construed as a potential conflict of interest.
Acknowledgments
We are grateful for skillful laboratory assistance by B. Christensen, K. C. Kirkegaard, and R. O. Holm, as well as several engaged students over the years.
Supplementary Material
The Supplementary Material for this article can be found online at: https://www.frontiersin.org/articles/10.3389/fmars.2021.649360/full#supplementary-material
References
Albrecht, A. S. (1998). Soft bottom versus hard rock: Community ecology of macroalgae on intertidal mussel beds in the Wadden Sea. J. Exp. Mar. Biol. Ecol. 229, 85–109. doi: 10.1016/s0022-0981(98)00044-6
Alestra, T., and Schiel, D. R. (2014). Effects of opportunistic algae on the early life history of a habitat-forming fucoid: influence of temperature, nutrient enrichment and grazing pressure. Mar. Ecol. Prog. Ser. 508, 105–115. doi: 10.3354/meps10838
Bartoli, M., Zilius, M., Bresciani, M., Vaiciute, D., Vybernaite-Lubiene, I., Petkuviene, J., et al. (2018). Drivers of cyanobacterial blooms in a hypertrophic lagoon. Front. Mar. Sci. 5:434. doi: 10.3389/fmars.2018.00434
Bittick, S. J., Sutula, M., and Fong, P. (2018). A tale of two algal blooms: Negative and predictable effects of two common bloom-forming macroalgae on seagrass and epiphytes. Mar. Environ. Res. 140, 1–9. doi: 10.1016/j.marenvres.2018.05.018
Björnsäter, B. R., and Wheeler, P. A. (1990). Effect of nitrogen and phosphorus supply on growth and tissue composition of Ulva fenestrata and Enteromorpha intestinalis (Ulvales, Chlorophyta). J. Phycol. 26, 603–611. doi: 10.1111/j.0022-3646.1990.00603.x
Bolhuis, H., Fillinger, L., and Stal, L. J. (2013). Coastal microbial mat diversity along a natural salinity gradient. PLoS One 8:63166. doi: 10.1371/journal.pone.0063166
Boorman, L. A., and Hazelden, J. (2017). Managed re-alignment; a salt marsh dilemma? Wetlands Ecol. Manage. 25, 387–403. doi: 10.1007/s11273-017-9556-9
Bosello, F., Nicholls, R. J., Richards, J., Roson, R., and Tol, R. S. J. (2012). Economic impacts of climate change in Europe: sea-level rise. Clim. Chang. 112, 63–81. doi: 10.1007/s10584-011-0340-1
Canal-Vergés, P., Potthoff, M., Hansen, F. T., Holmboe, N., Rasmussen, E. K., and Flindt, M. R. (2014). Eelgrass re-establishment in shallow estuaries is affected by drifting macroalgae – Evaluated by agent-based modeling. Ecol. Model. 272, 116–128. doi: 10.1016/j.ecolmodel.2013.09.008
Carey, J. (2020). Managed retreat increasingly seen as necessary in response to climate change’s fury. Proc. Natl. Acad. Sci. 117, 13182–13185. doi: 10.1073/pnas.2008198117
Carreiro-Silva, M., Kiene, W. E., Golubic, S., and McClanahan, T. R. (2012). Phosphorus and nitrogen effects on microbial euendolithic communities and their bioerosion rates. Mar. Pollut. Bull. 64, 602–613. doi: 10.1016/j.marpolbul.2011.12.013
Clayton, M. (1992). Propagules of marine macroalgae, structure and development. Br. Phycol. J. 27, 219–232. doi: 10.1080/00071619200650231
Dale, J., Cundy, A. B., Spencer, K. L., Carr, S. J., Croudace, I. W., Burgess, H. M., et al. (2019). Sediment structure and physicochemical changes following tidal inundation at a large open coast managed realignment site. Sci. Tot. Environ. 660, 1419–1432. doi: 10.1016/j.scitotenv.2018.12.323
Derolez, V., Malet, N., Fiandrino, A., Lagarde, F., Richard, M., Ouisse, V., et al. (2020). Fifty years of ecological changes: Regime shifts and drivers in a coastal Mediterranean lagoon during oligotrophication. Sci. Tot. Env. 732:139292. doi: 10.1016/j.scitotenv.2020.139292
Esteves, L. S. (2014). “What is Managed Realignment?,” in Managed realignment: A viable long-term coastal management strategy?, ed. L. S. Esteves (Dordrecht: Springer Briefs in Environmental Science), 19–28. doi: 10.1007/978-94-017-9029-1_2
Fong, C. R., and Fong, P. (2018). Nutrient fluctuations in marine systems: Press versus pulse nutrient subsidies affect producer competition and diversity in estuaries and coral reefs. Estuar. Coast. 41, 421–429. doi: 10.1007/s12237-017-0291-5
Fong, P., and Zedler, J. B. (1993). Temperature and light effects on the seasonal succession of algal communities in shallow coastal lagoons. J. Exp. Mar. Biol. Ecol. 171, 259–272. doi: 10.1016/0022-0981(93)90008-c
Fong, P., Donohoe, R. M., and Zedler, J. B. (1993a). Competition with macroalgae and benthic cyanobacterial mats limits phytoplankton abundance in experimental microcosms. Mar. Ecol. Prog. Ser. 100, 97–102. doi: 10.3354/meps100097
Fong, P., Zedler, J. B., and Donohoe, R. M. (1993b). Nitrogen vs phosphorus limitation of algal biomass in shallow coastal lagoons. Limnol. Oceanog. 38, 906–923. doi: 10.4319/lo.1993.38.5.0906
Gagnon, K., Sjöroos, J., Yli-Rosti, J., Stark, M., Rothäusler, E., and Jormalainen, V. (2016). Nutrient enrichment overwhelms top-down control in algal communities around cormorant colonies. J. Exp. Mar. Biol. Ecol. 476, 31–40. doi: 10.1016/j.jembe.2015.12.007
García-Robledo, E., and Corzo, A. (2011). Effects of macroalgal blooms on carbon and nitrogen biogeochemical cycling in photoautotrophic sediments: an experimental mesocosm. Mar. Pollut. Bull. 62, 1550–1556. doi: 10.1016/j.marpolbul.2011.03.044
Gardner, W. S., Seitzinger, S. P., and Malczyk, J. M. (1991). The effects of sea salts on the forms of nitrogen released from estuaries and freshwater sediments: Does ion pairing affect ammonium flux? Estuaries 14, 157–166. doi: 10.2307/1351689
Gerwing, T. G., Davies, M. M., Clements, J., Flores, A.-M., Thomson, H. M., Nelson, K. R., et al. (2020). Do you want to breach an embankment? Synthesis of the literature and practical considerations for breaching of tidally influenced causeways and dikes. Estuar. Coast. Shelf Sci. 245:107024. doi: 10.1016/j.ecss.2020.107024
Gladyshev, M. I., and Gubelit, Y. I. (2019). Green tides: New consequences of the eutrophication of natural waters (invited review). Contemp. Probl. Ecol. 12, 109–125. doi: 10.1134/s1995425519020057
Green, L., Sutula, M., and Fong, P. (2014). How much is too much? Identifying benchmarks of adverse effects of macroalgae on the macrofauna in intertidal flats. Ecol. Appl. 24, 300–314. doi: 10.1890/13-0524.1
Guldberg, L. B., Finster, K., Jørgensen, N. O. G., Middelboe, M., and Lomstein, B. (2002). Utilization of marine sedimentary dissolved organic nitrogen by native anaerobic bacteria. Limnol. Oceanogr. 47, 1712–1722. doi: 10.4319/lo.2002.47.6.1712
Hinkel, J., Lincke, D., Vafeidis, A. T., Perrette, M., Nicholls, R. J., Tol, R. S. J., et al. (2014). Coastal flood damage and adaptation costs under 21st century sea-level rise. Proc. Natl. Acad. Sci. 111, 3292–3297. doi: 10.1073/pnas.1222469111
Howarth, R. W., and Marino, R. (2006). Nitrogen as the limiting nutrient for eutrophication in coastal marine ecosystems: Evolving views over three decades. Limnol. Oceanog. 51, 364–376. doi: 10.4319/lo.2006.51.1_part_2.0364
Høgslund, S., Dahl, K., Krause-Jensen, D., Lundsteen, S., Rasmussen, M. B., and Windelin, A. (2014). Makroalger på Kystnær Hårdbund (in Danish). Tenisk Anvisning M12. Aarhus: DCE, Aarhus University.
Hughes, R. G., Fletcher, P. W., and Hardy, M. J. (2009). Successional development of saltmarsh in two managed realignment areas in SE England, and prospects for saltmarsh restoration. Mar. Ecol. Prog. Ser. 384, 13–22. doi: 10.3354/meps08027
IPCC (2019). IPCC Special Report on the Ocean and Cryosphere in a Changing Climate. Geneva: Intergovernmental Panel on Climate Change.
Kim, S., Park, S. R., Kang, Y. H., Kim, G.-Y., Lee, K.-S., Lee, H. J., et al. (2014). Usefulness of tissue nitrogen content and macroalgal community structure as indicators of water eutrophication. J. Appl. Phycol. 26, 1149–1158. doi: 10.1007/s10811-013-0194-1
Koch, E. W. (2001). Beyond light: Physical, geological, and geochemical parameters as possible submersed aquatic vegetation habitat requirements. Estuaries 24, 1–17. doi: 10.2307/1352808
Koroleff, F. (1983). “Determination of phosphorus,” in Methods of seawater analysis, eds K. Grashof, M. Erhardt, and K. Kremling (Weinheim: Verlag Chemie), 125–131.
Korpinen, S., Honkanen, T., Vesakoski, O., Hemmi, A., Koivikko, R., Loponen, J., et al. (2007). Macroalgal communities face the challenge of changing biotic interactions: Review with focus on the Baltic Sea. Ambio 36, 203–211. doi: 10.1579/0044-7447(2007)36[203:mcftco]2.0.co;2
Kraal, P., Burton, E., Rose, A. L., Kocar, B. D., Lockhart, R. S., Grice, K., et al. (2015). Sedimentary iron-phosphorus cyclin under contrasting redox conditions in eutrophic estuary. Chem. Geol. 392, 19–31. doi: 10.1016/j.chemgeo.2014.11.006
Kraufvelin, P. (2007). Responses to nutrient enrichment, wave action and disturbance in rocky shore communities. Aquat. Bot. 87, 62–274.
Kraufvelin, P., Ruuskanen, A. T., Nappu, N., and Kiirikki, M. (2007). Winter colonisation and succession of filamentous macroalgae on artificial substrates and possible relationships to Fucus vesiculosus settlement in early summer. Estuar. Coast. Shelf Sci. 72, 665–674. doi: 10.1016/j.ecss.2006.11.029
Kristensen, E., Quintana, C. O., Valdemarsen, T., and Flindt, M. R. (2020). Nitrogen and phosphorus export after flooding of agricultural land by coastal managed realignment. Estuar. Coast. 2020:2. doi: 10.1007/s12237-020-00785-2
Ladakis, M., Dassenakis, M., and Pantazidou, A. (2006). Nitrogen and phosphorus in coastal sediments covered by cyanobacteria mats. J. Soil Sed. 6, 46–54. doi: 10.1065/jss2005.10.150
Le Fur, I., De Wit, R., Plus, M., Oheix, J., Derolez, V., Simier, M., et al. (2019). Re-oligotrophication trajectories of macrophyte assemblages in Mediterranean coastal lagoons based on 17-year time-series. Mar. Ecol. Prog. Ser. 608, 13–32. doi: 10.3354/meps12814
Littler, M. M., Littler, D. S., and Brooks, B. L. (2010). The effects of nitrogen and phosphorus enrichment on algal community development: Artificial mini-reefs on the Belize Barrier Reef sedimentary lagoon. Harmful Algae 9, 255–263. doi: 10.1016/j.hal.2009.11.002
Lotze, H. K., and Schramm, W. (2000). Ecophysiological traits explains species dominance patterns in macroalgal blooms. J. Phycol. 295, 287–295. doi: 10.1046/j.1529-8817.2000.99109.x
Lotze, H. K., Worm, B., and Sommer, U. (2001). Strong bottom-up and top-down control of early life stages of macroalgae. Limnol. Oceanog. 46, 749–757. doi: 10.4319/lo.2001.46.4.0749
Maie, N., Parish, K. J., Watanabe, A., Knicker, H., Benner, R., Abe, T., et al. (2006). Chemical characteristics of dissolved organic nitrogen in an oligotrophic subtropical coastal ecosystem. Geochim. Cosmochim. Acta 70, 4491–4506. doi: 10.1016/j.gca.2006.06.1554
Malm, T., Kautsky, L., and Claesson, T. (2003). The density and survival of Fucus vesiculosus L. (Fucales, Phaeophyta) on different bedrock types on a Baltic Sea moraine coast. Bot. Mar. 46, 256–262.
Marino, R., Howarth, R. W., Chan, F., Cole, J. J., and Likens, G. E. (2003). Sulfate inhibition of molybdenum-dependent nitrogen fixation by planktonic cyanobacteria under seawater conditions: A non-reversible effect. Hydrobiologia 500, 277–293. doi: 10.1023/a:1024641904568
Marks, L. M., Reed, D. C., and Holbrook, S. J. (2020). Niche complementarity and resistance to grazing promote the invasion success of Sargassum horneri in North America. Diversity 12:d12020054. doi: 10.3390/d12020054
Martínez, B., Pato, L. S., and Rico, J. M. (2012). Nutrient uptake and growth responses of three intertidal macroalgae with perennial, opportunistic and summer-annual strategies. Aquat. Bot. 96, 14–22. doi: 10.1016/j.aquabot.2011.09.004
Matthiesen, H., Leipe, T., and Laima, M. J. C. (2001). A new experimental setup for studying the formation of phosphate binding iron oxides in marine sediments. Preliminary results. Biogeochemistry 52, 79–92.
McGovern, J. V., Nash, S., and Hartnett, M. (2019). Interannual improvement in sea lettuce blooms in an agricultural catchment. Front. Mar. Sci. 6:64. doi: 10.3389/fmars.2019.00064
Middelboe, A. L., and Sand-Jensen, K. (2000). Long-term changes in macroalgal communities in a Danish estuary. Phycologia 39, 245–257. doi: 10.2216/i0031-8884-39-3-245.1
Mossman, H. L., Brown, M. J. H., Davy, A., and Grant, A. (2012). Managed coastal realignment: dispersal limitation or environmental tolerance? Restor. Ecol. 20, 65–75. doi: 10.1111/j.1526-100x.2010.00745.x
Nejrup, L. B., and Pedersen, M. F. (2012). The effect of temporal variability in salinity on the invasive red alga Gracilaria vermiculophylla. Eur. J. Phycol. 47, 254–263. doi: 10.1080/09670262.2012.702225
Nejrup, L. B., Stæhr, P. A., and Thomsen, M. S. (2013). Temperature- and light-dependent growth and metabolism of the invasive red algae Gracilaria vermiculophylla - a comparison with two native macroalgae. Eur. J. Phycol. 48, 295–308. doi: 10.1080/09670262.2013.830778
Nicholls, R. J., and Cazenave, A. (2010). Sea-level rise and its impact on coastal zones. Science 328, 1517–1520. doi: 10.1126/science.1185782
Nordstrom, K. F., Jackson, N. L., and Roman, C. T. (2016). Facilitation landform migration by removing shore protection structures: Opportunities and constraints. Environ. Sci. Pol. 66, 217–226. doi: 10.1016/j.envsci.2016.07.012
Nyberg, C. D., Thomsen, M. S., and Wallentinus, I. (2009). Flora and fauna associated with the introduced red alga Gracilaria vermiculophylla. Eur. J. Phycol. 44, 395–403. doi: 10.1080/09670260802592808
Parsons, T. R., Maita, Y., and Lalli, C. M. (1984). A manual of Chemical and biological methods for seawater analysis. Oxford: Pergamon Press.
Paterson, D. M., and Hagerthey, S. E. (2001). “Microphytobenthos in contrasting coastal ecosystems: Biology and dynamics,” in Sandy and muddy shores: ecological comparisons, ed. K. Reise (Berlin: Springer-Verlag), 105–126. doi: 10.1007/978-3-642-56557-1_6
Pedersen, M. F., and Borum, J. (1996). Nutrient control of algal growth in estuarine waters. Nutrient limitation and the importance of nitrogen requirements and nitrogen storage among phytoplankton and species of macroalgae. Mar. Ecol. Prog. Ser. 142, 261–272. doi: 10.3354/meps142261
Pedersen, M. F., and Borum, J. (1997). Nutrient control of estuarine macroalgae: Growth strategy and the balance between nitrogen requirements and uptake. Mar. Ecol. Prog. Ser. 161, 155–163. doi: 10.3354/meps161155
Pedersen, M. F., and Johnsen, K. L. (2017). Nutrient (N and P) dynamics of the invasive macroalga Gracilaria vermiculophylla: nutrient uptake kinetics and nutrient release through decomposition. Mar. Biol. 64, 1–12.
Pedersen, M. F., Borum, J., and Fotel, F. L. (2010). Phosphorus dynamics and limitation of fast-and slow-growing temperate seaweeds in Oslofjord, Norway. Mar. Ecol. Prog. Ser. 399, 103–115. doi: 10.3354/meps08350
Pinckney, J., Paerl, H. W., and Fitzpatrick, M. (1995). Impact of seasonality and nutrients on microbial mat community structure and function. Mar. Ecol. Prog. Ser. 123, 207–216. doi: 10.3354/meps123207
Pinedo, S., Zabala, M., and Ballesteros, E. (2013). Long-term changes in sublittoral macroalgal assemblages related to water quality improvement. Bot. Mar. 56, 461–469.
Raffaelli, D., and Hawkins, S. (1999). Intertidal Ecology, 2nd Edn. Dordrecht: Kluwer Academic Publishers.
Rasmussen, J. R., Dromph, K. M., Göke, C., and Krause-Jensen, D. (2015). Reduced cover of drifting macroalgae following nutrient reduction in Danish coastal waters. Estuar. Coast. 38, 1664–1677. doi: 10.1007/s12237-014-9904-4
Raven, J. A., and Taylor, R. (2002). Macroalgal growth in nutrient-enriched estuaries. Water Air Soil Pollut. 3, 7–26.
Rossi, F., and De Philippis, R. (2015). Role of cyanobacterial exopolysaccharides in phototrophic biofilms and in complex microbial mats. Life 5, 1218–1238. doi: 10.3390/life5021218
Rubæk, G. H., Kristensen, K., Olesen, S. E., Østergaard, H. S., and Heckrath, G. (2013). Phosphorus accumulation and spatial distribution in agricultural soils in Denmark. Geoderma 209-210, 241–250. doi: 10.1016/j.geoderma.2013.06.022
Rysgaard, S., Thastum, P., Dalsgaard, T., Christensen, P. B., and Sloth, N. P. (1999). Effects of salinity on NH4+ adsorption capacity, nitrification, and denitrification in Danish estuarine sediments. Estuaries 22, 21–30. doi: 10.2307/1352923
Schagerström, E., and Kautsky, L. (2016). Despite marine traits, the endemic Fucus radicans (Phaeophyceae) is restricted to the brackish Baltic Sea. Eur. J. Phycol. 51, 378–386. doi: 10.1080/09670262.2016.1183234
Sebilo, M., Mayer, B., Nicolardot, B., Pinay, G., and Mariotti, A. (2013). Long-term fate of nitrate fertilizer in agricultural soils. Proc. Natl. Acad. Sci. 110, 18185–18189. doi: 10.1073/pnas.1305372110
Shpigel, M., Guttman, L., Ben-Ezra, D., Yu, J., and Chen, S. (2019). Is Ulva sp. able to be an efficient biofilter for mariculture effluents? J. Appl. Phycol. 31, 2449–2459. doi: 10.1007/s10811-019-1748-7
Sjøgaard, K. S., Valdemarsen, T., and Treusch, A. H. (2018). Responses of an agricultural soil microbiome to flooding with seawater after managed coastal realignment. Microorganisms 6:6010012. doi: 10.3390/microorganisms6010012
Sousa, W. P. (1979). Experimental investigations of disturbance and ecological succession in a rocky intertidal algal community. Ecol. Monogr. 49, 227–254. doi: 10.2307/1942484
South, G. R., and Burrows, E. M. (1967). Studies on marine algae of the British Isles. 5. Chorda filum (L.) Stackh. Br. Phycol. Bull. 3, 379–402. doi: 10.1080/00071616700650261
Stal, L. J. (1995). Physiological ecology of cyanobacteria in microbial mats and other communities. N. Phytol. 131, 1–32. doi: 10.1111/j.1469-8137.1995.tb03051.x
Stal, L. J. (2001). Coastal microbial mats: the physiology of a small-scale ecosystem. S. Afr. J. Bot. 67, 399–410. doi: 10.1016/s0254-6299(15)31156-x
Stuart, R., Mayali, X., Lee, J. Z., Everroad, R. C., Hwang, M., Bebout, B. M., et al. (2016). Cyanobacterial reuse of extracellular organic carbon in microbial mats. ISME J. 10, 1240–1251. doi: 10.1038/ismej.2015.180
Sullivan, M. J. P., Davy, A. J., Grant, A., and Mossman, H. L. (2017). Is saltmarsh restoration success constrained by matching natural environments or altered succession? A test using niche models. J. Appl. Ecol. 55, 1207–1217. doi: 10.1111/1365-2664.13033
Sundbäck, K., Miles, A., Hulth, S., Pihl, L., Engström, P., Selander, E., et al. (2003). Importance of benthic nutrient regeneration during initiation of macroalgal blooms in shallow bays. Mar. Ecol. Prog. Ser. 246, 115–126. doi: 10.3354/meps246115
Sundby, B., Gobeil, C., Silverberg, N., and Mucci, A. (1992). The phosphorus cycle in marine sediments. Limnol. Oceanog. 37, 1129–1145. doi: 10.4319/lo.1992.37.6.1129
Tabak, M. A., Webb, C. T., and Miller, R. S. (2018). Propagule size and structure, life history, and environmental conditions affect establishment success of an invasive species. Sci. Rep. 8, 1–9.
Taylor, R., Fletcher, R. L., and Raven, J. A. (2001). Preliminary studies on the growth of selected ‘green tide’ algae in laboratory culture: Effects of irradiance, temperature, salinity and nutrients on growth rate. Bot. Mar. 44, 327–336. doi: 10.2216/i0031-8884-35-4-327.1
Teichberg, M., Fox, S. E., Olsen, Y. S., Valiela, I., Martinetto, P., Iribarne, O., et al. (2010). Eutrophication and macroalgal blooms in temperate and tropical coastal waters: Nutrient enrichment experiments with Ulva spp. Glob. Chang. Biol. 16, 2624–2637. doi: 10.1111/j.1365-2486.2009.02108.x
Thacker, R., and Paul, V. (2001). Are benthic cyanobacteria indicators of nutrient enrichment? Relationships between cyanobacterial abundance and environmental factors on the reef flats of Guam. Bull. Mar. Sci. 69, 497–508.
Thomsen, M. S., Stæhr, P., Nyberg, C. D., Krause-Jensen, D., Schwærter, S., and Silliman, B. (2007a). Gracilaria vermiculophylla in northern Europe, with focus on Denmark, and what to expect in the future. Aquat. Invas. 2, 83–94. doi: 10.3391/ai.2007.2.2.1
Thomsen, M. S., Wernberg, T., Stæhr, P., Krause-Jensen, D., Risgaard-Petersen, N., and Silliman, B. R. (2007b). Alien macroalgae in Denmark - A broad-scale national perspective. Mar. Biol. Res. 3, 61–72. doi: 10.1080/17451000701213413
Thorsen, S. W., Kristensen, E., Valdemarsen, T., Flindt, M. R., Quintana, C. O., and Holmer, M. (2019). Fertilizer-derived N in opportunistic macroalgae after flooding of agricultural land. Mar. Ecol. Prog. Ser. 616, 37–49. doi: 10.3354/meps12927
Tyler, A. C., McGlathery, K. J., and Anderson, I. C. (2001). Macroalgae mediation of dissolved organic nitrogen fluxes in a temperate coastal lagoon. Estuar. Coast. Shelf Sci. 53, 155–168. doi: 10.1006/ecss.2001.0801
Valdemarsen, T., Canal-Vergés, P., Kristensen, E., Holmer, M., Kristiansen, M. D., and Flindt, M. R. (2010). Vulnerability of Zostera marina seedlings to physical stress. Mar. Ecol. Prog. Ser. 418, 119–130. doi: 10.3354/meps08828
Valdemarsen, T., Quintana, C. O., Thorsen, S. W., and Kristensen, E. (2018). Benthic macrofauna bioturbation and early colonization in newly flooded coastal habitats. PLoS One 13:196097. doi: 10.1371/journal.pone.0196097
Valiela, I. (1984). “The structure of marine communities over time: Colonization and Succession,” in Marine Ecological Processes, ed. D. E. Reichle (New York: Springer Science), 547.
Valiela, I., Mcclelland, J., Hauxwell, J., Behr, P. J., Hersh, D., and Foreman, K. (1997). Macroalgal blooms in shallow estuaries: controls and ecophysiological and ecosystem consequences. Limnol. Oceanog. 42, 1105–1118. doi: 10.4319/lo.1997.42.5_part_2.1105
Van Alstyne, K. L., McCarthy, J. J. III, Hustead, C. L., and Duggins, D. O. (1999). Geographic variation in polyphenolic levels of Northeastern Pacific kelps and rockweeds. Mar. Biol. 133, 371–379. doi: 10.1007/s002270050476
Van Engeland, T., Bouma, T. J., Morris, E. P., Brun, F. G., Peralta, G., Lara, M., et al. (2013). Dissolved organic matter uptake in a temperate seagrass ecosystem. Mar. Ecol. Prog. Ser. 478, 87–100. doi: 10.3354/meps10183
Wallentinus, I. (1984). Comparisons of nutrient uptake rates for Baltic macroalgae with different thallus morphologies. Mar. Biol. 80, 215–225. doi: 10.1007/bf02180189
Warner, J. F., van Staveren, M. F., and van Tatenhove, J. (2018). Cutting dikes, cutting ties? Reintroducing flood dynamics in coastal polders in Bangladesh and the Netherlands. Internat. J. Disast. Risk Reduct. 32, 106–112. doi: 10.1016/j.ijdrr.2018.03.020
Wells, E., Wilkinson, M., Wood, P., and Scanlan, C. (2007). The use of macroalgal species richness and composition on intertidal rocky seashores in the assessment of ecological quality under the European Water Framework Directive. Mar. Pollut. Bull. 55, 151–161. doi: 10.1016/j.marpolbul.2006.08.031
Wikström, S. A., Carstensen, J., Blomqvist, M., and Krause-Jensen, D. (2016). Cover of coastal vegetation as an indicator of eutrophication along environmental gradients. Mar. Biol. 163:257. doi: 10.1007/s00227-016-3032-6
Keywords: coastal restoration, managed realignment, agricultural soil, macroalgae, cyanobacteria, internal loading, DIN:DIP ratio
Citation: Thorsen SW, Holmer M, Quintana CO, Valdemarsen T and Kristensen E (2021) Internal Nutrient Loading Controls Macroalgal and Cyanobacterial Succession in a Coastal Lagoon Restored by Managed Realignment of Agricultural Land. Front. Mar. Sci. 8:649360. doi: 10.3389/fmars.2021.649360
Received: 04 January 2021; Accepted: 10 March 2021;
Published: 30 March 2021.
Edited by:
Dongyan Liu, East China Normal University, ChinaReviewed by:
Xuechu Chen, East China Normal University, ChinaKeqiang Li, Ocean University of China, China
Copyright © 2021 Thorsen, Holmer, Quintana, Valdemarsen and Kristensen. This is an open-access article distributed under the terms of the Creative Commons Attribution License (CC BY). The use, distribution or reproduction in other forums is permitted, provided the original author(s) and the copyright owner(s) are credited and that the original publication in this journal is cited, in accordance with accepted academic practice. No use, distribution or reproduction is permitted which does not comply with these terms.
*Correspondence: Erik Kristensen, ebk@biology.sdu.dk